4 Igneous Processes and Volcanoes
Learning Objectives
By the end of this chapter, students should be able to:
- Explain the origin of magma as it relates to plate tectonics.
- Describe how the Bowen’s Reaction Series relates mineral crystallization and melting temperatures.
- Explain how cooling of magma leads to rock compositions and textures, and how these are used to classify igneous rocks.
- Analyze the features of common igneous landforms and how they relate to their origin.
- Describe how silica content affects magma viscosity and eruptive style of volcanoes.
- Describe volcano types, eruptive styles, composition, and their plate tectonic settings.
- Describe volcanic hazards.
Igneous rock is formed when liquid rock freezes into a solid rock. This molten material is called magma when it is in the ground and lava when it is on the surface. Only the Earth’s outer core is liquid; the Earth’s mantle and crust is naturally solid. However, there are a few minor pockets of magma that form near the surface where geologic processes cause melting. It is this magma that becomes the source for volcanoes and igneous rocks. This chapter will describe the classification of igneous rocks, the unique processes that form magmas, types of volcanoes and volcanic processes, volcanic hazards, and igneous landforms.
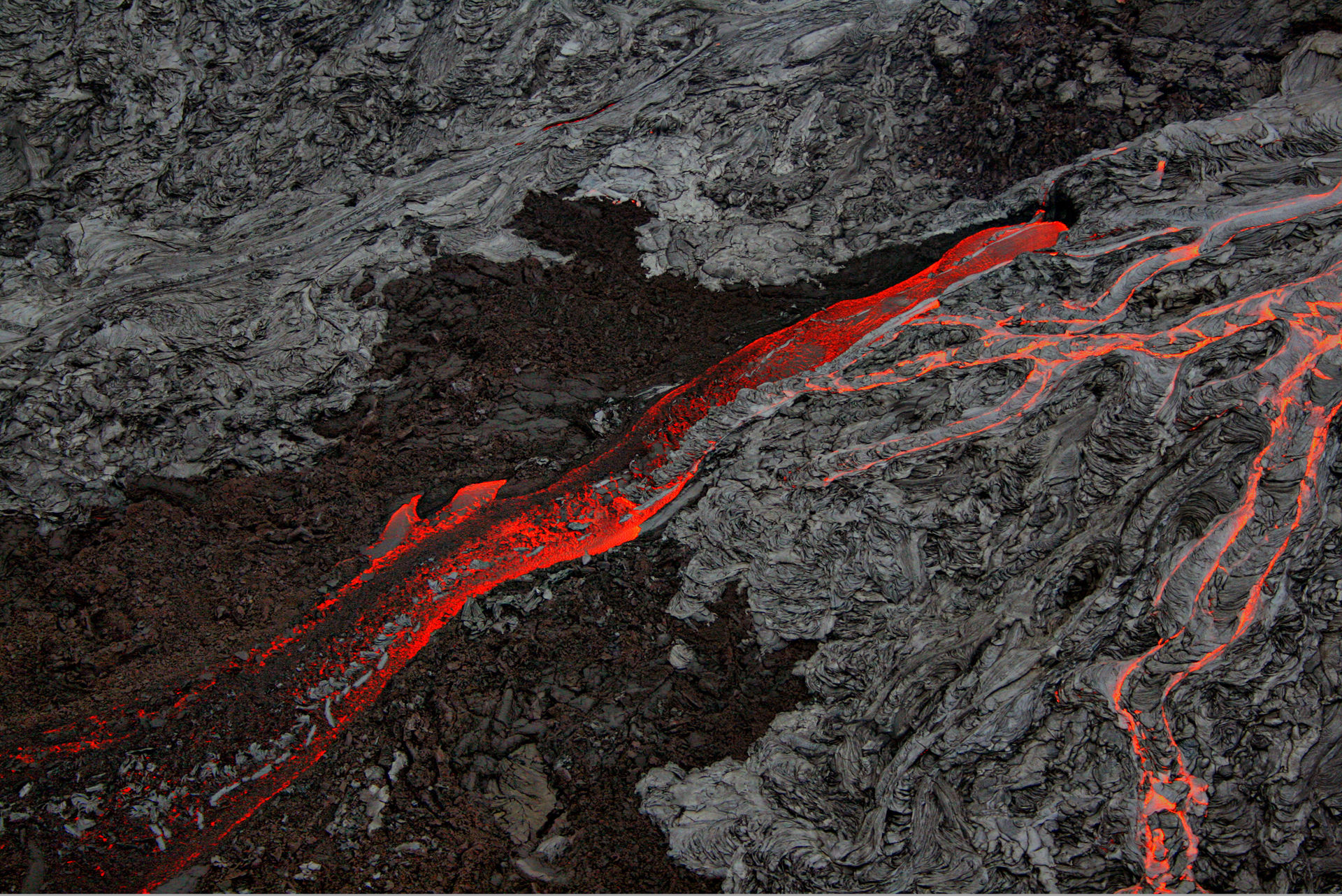
Lava cools quickly on the surface of the earth and forms tiny microscopic crystals. These are known as fine-grained extrusive, or volcanic, igneous rocks. Extrusive rocks are often vesicular, filled with holes from escaping gas bubbles. Volcanism is the process in which lava is erupted. Depending on the properties of the lava that is erupted, the volcanism can be drastically different, from smooth and gentle to dangerous and explosive. This leads to different types of volcanoes and different volcanic hazards.
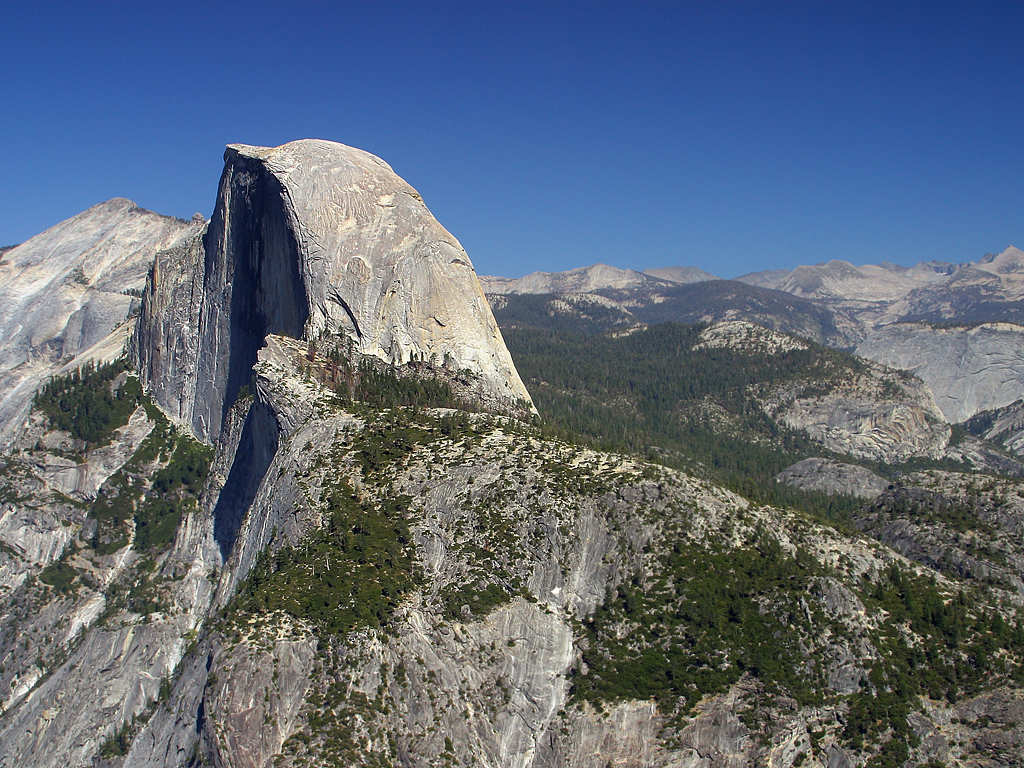
In contrast, magma that cools slowly below the earth’s surface forms larger crystals which can be seen with the naked eye. These are known as coarse-grained intrusive, or plutonic, igneous rocks. This relationship between cooling rates and grain sizes of the solidified minerals in igneous rocks is important for interpreting the rock’s geologic history.
4.1 Classification of Igneous Rocks
Igneous rocks are classified based on texture and composition. Texture describes the physical characteristics of the minerals, such as grain size. This relates to the cooling history of the molten magma from which it came. Composition refers to the rock’s specific mineralogy and chemical composition. Cooling history is also related to changes that can occur to the composition of igneous rocks.
4.1.1 Texture
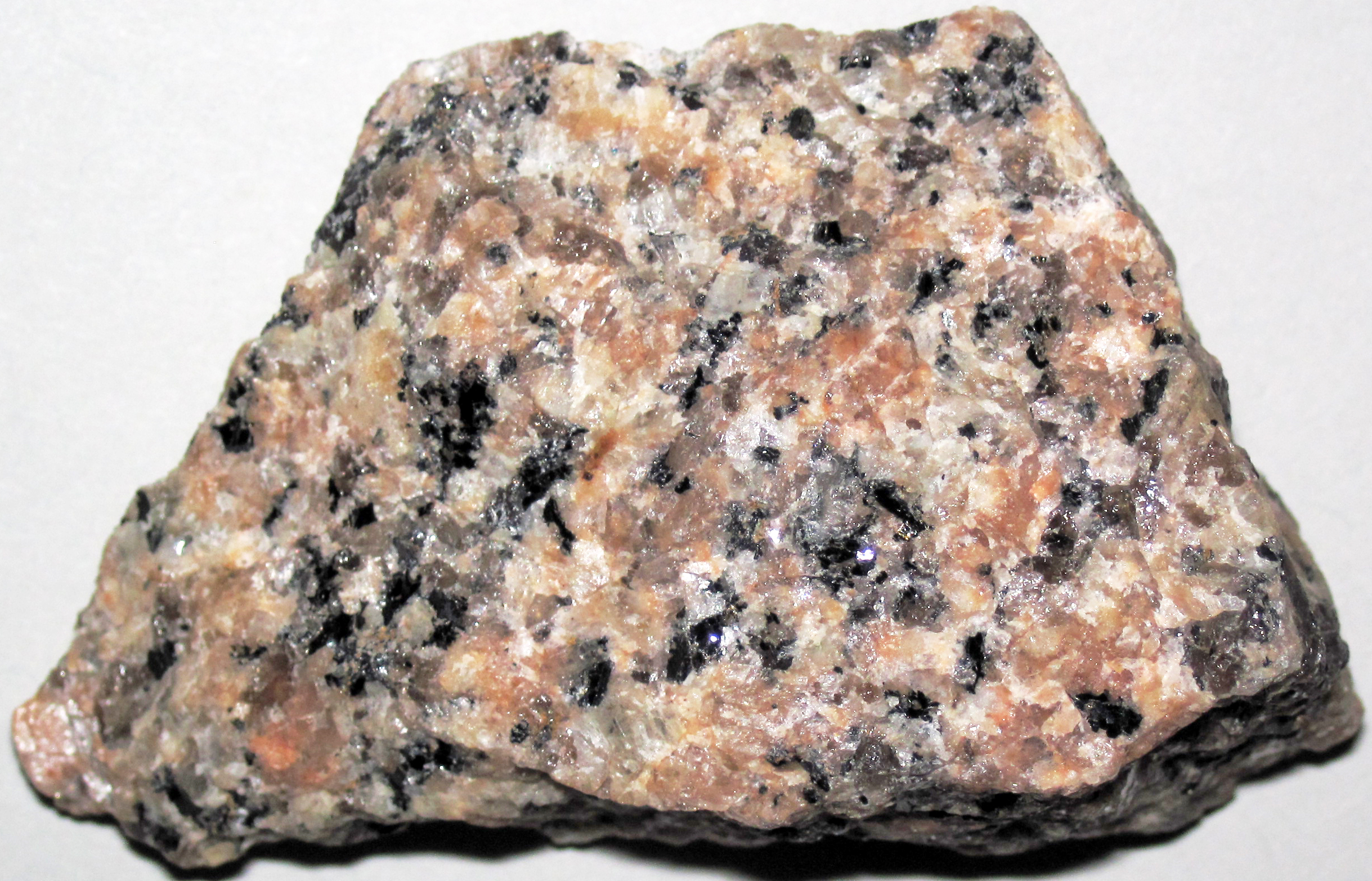
If magma cools slowly, deep within the crust, the resulting rock is called intrusive or plutonic. The slow cooling process allows crystals to grow large, giving intrusive igneous rock a coarse-grained or phaneritic texture. The individual crystals in phaneritic texture are readily visible to the unaided eye.
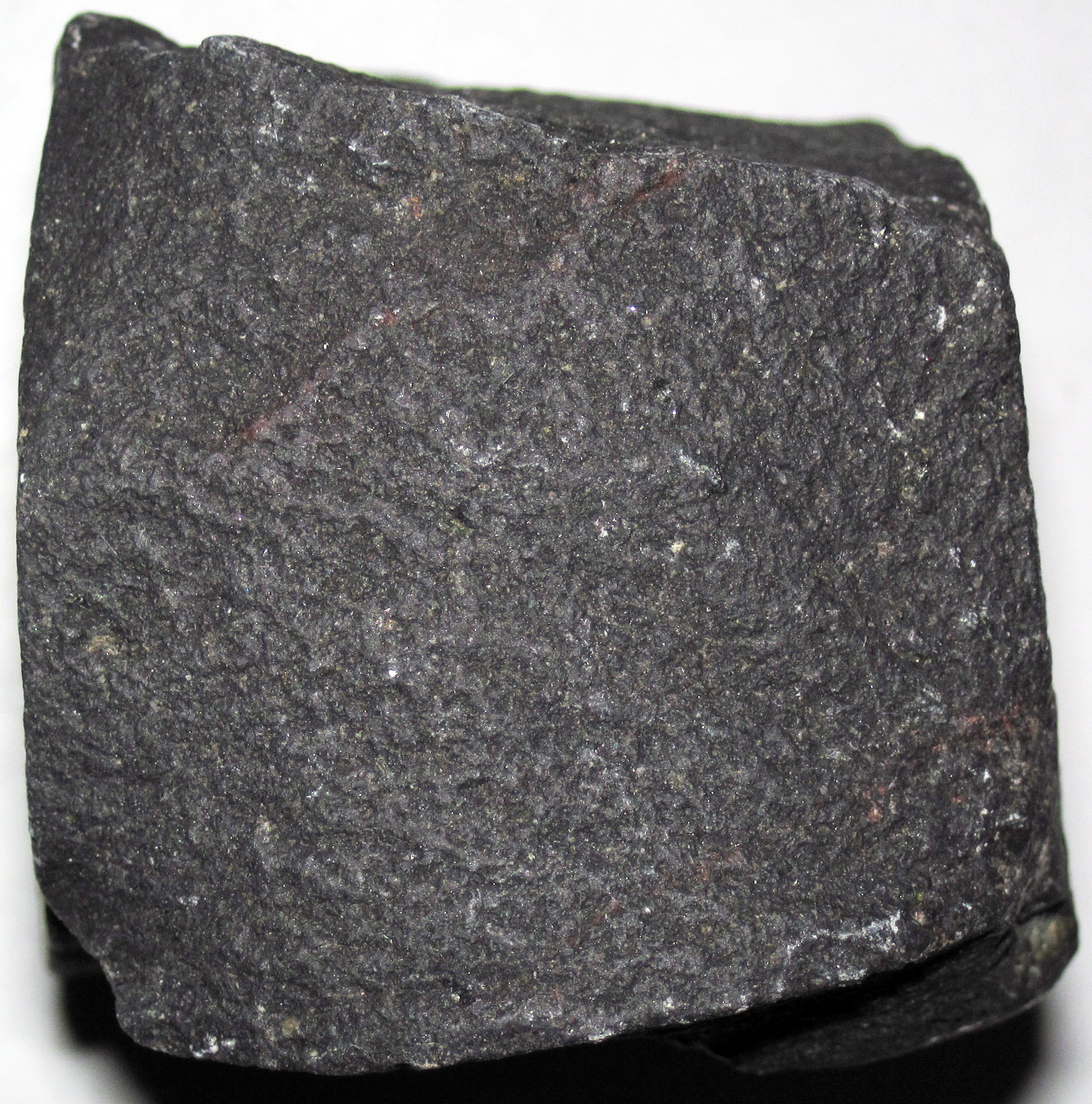
When lava is extruded onto the surface, or intruded into shallow fissures near the surface and cools, the resulting igneous rock is called extrusive or volcanic. Extrusive igneous rocks have a fine-grained or aphanitic texture, in which the grains are too small to see with the unaided eye. The fine-grained texture indicates the quickly cooling lava did not have time to grow large crystals. These tiny crystals can be viewed under a petrographic microscope. In some cases, extrusive lava cools so rapidly it does not develop crystals at all. This non-crystalline material is not classified as minerals, but as volcanic glass. This is a common component of volcanic ash and rocks like obsidian.
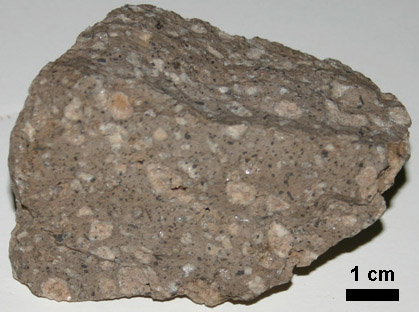
Some igneous rocks have a mix of coarse-grained minerals surrounded by a matrix of fine-grained material in a texture called porphyritic. The large crystals are called phenocrysts and the fine-grained matrix is called the groundmass or matrix. Porphyritic texture indicates the magma body underwent a multi-stage cooling history, cooling slowly while deep under the surface and later rising to a shallower depth or the surface where it cooled more quickly.
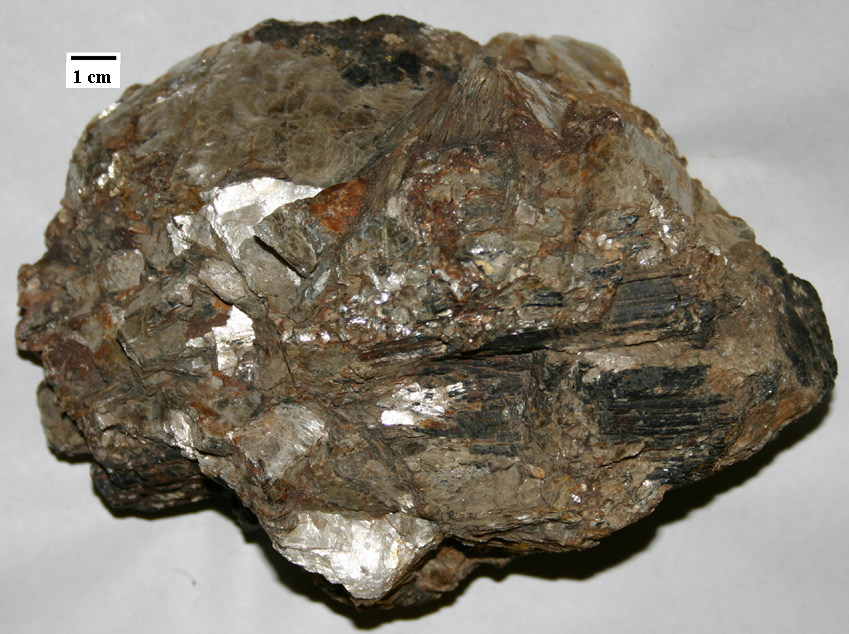
Residual molten material expelled from igneous intrusions may form veins or masses containing very large crystals of minerals like feldspar, quartz, beryl, tourmaline, and mica. This texture, which indicates a very slow crystallization, is called pegmatitic. A rock that chiefly consists of pegmatitic texture is known as a pegmatite. To give an example of how large these crystals can get, transparent cleavage sheets of pegmatitic muscovite mica were used as windows during the Middle Ages.
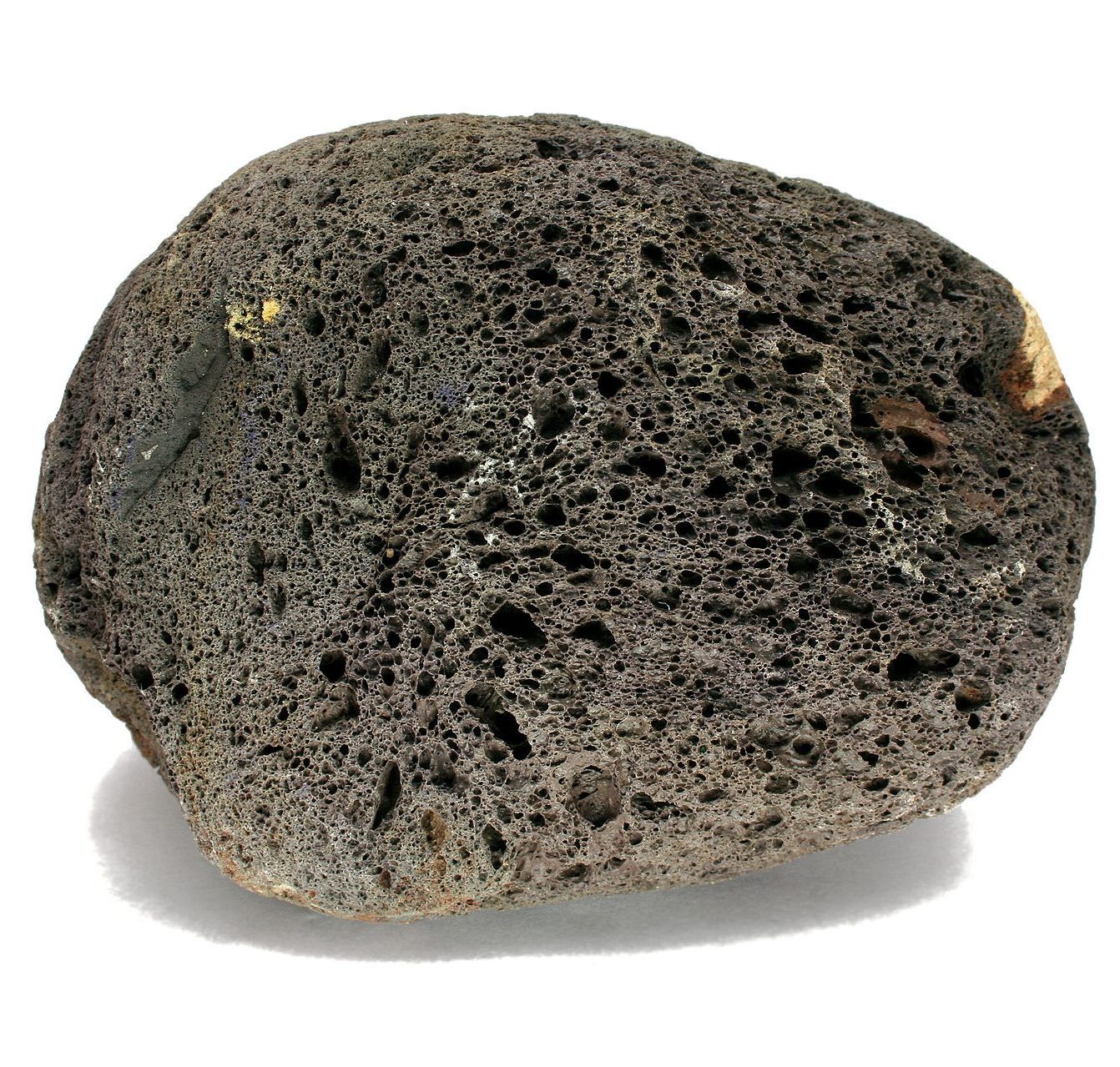
All magmas contain gases dissolved in solution called volatiles. As the magma rises to the surface, the drop in pressure causes the dissolved volatiles to come bubbling out of solution, like the fizz in an opened bottle of soda. The gas bubbles become trapped in the solidifying lava to create a vesicular texture, with the holes specifically called vesicles. The type of volcanic rock with common vesicles is called scoria.
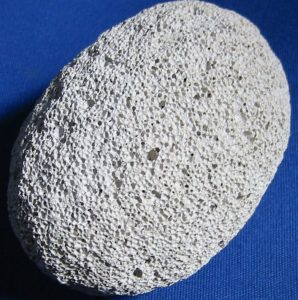
An extreme version of scoria occurs when volatile-rich lava is very quickly quenched and becomes a meringue-like froth of glass called pumice. Some pumice is so full of vesicles that the density of the rock drops low enough that it will float.
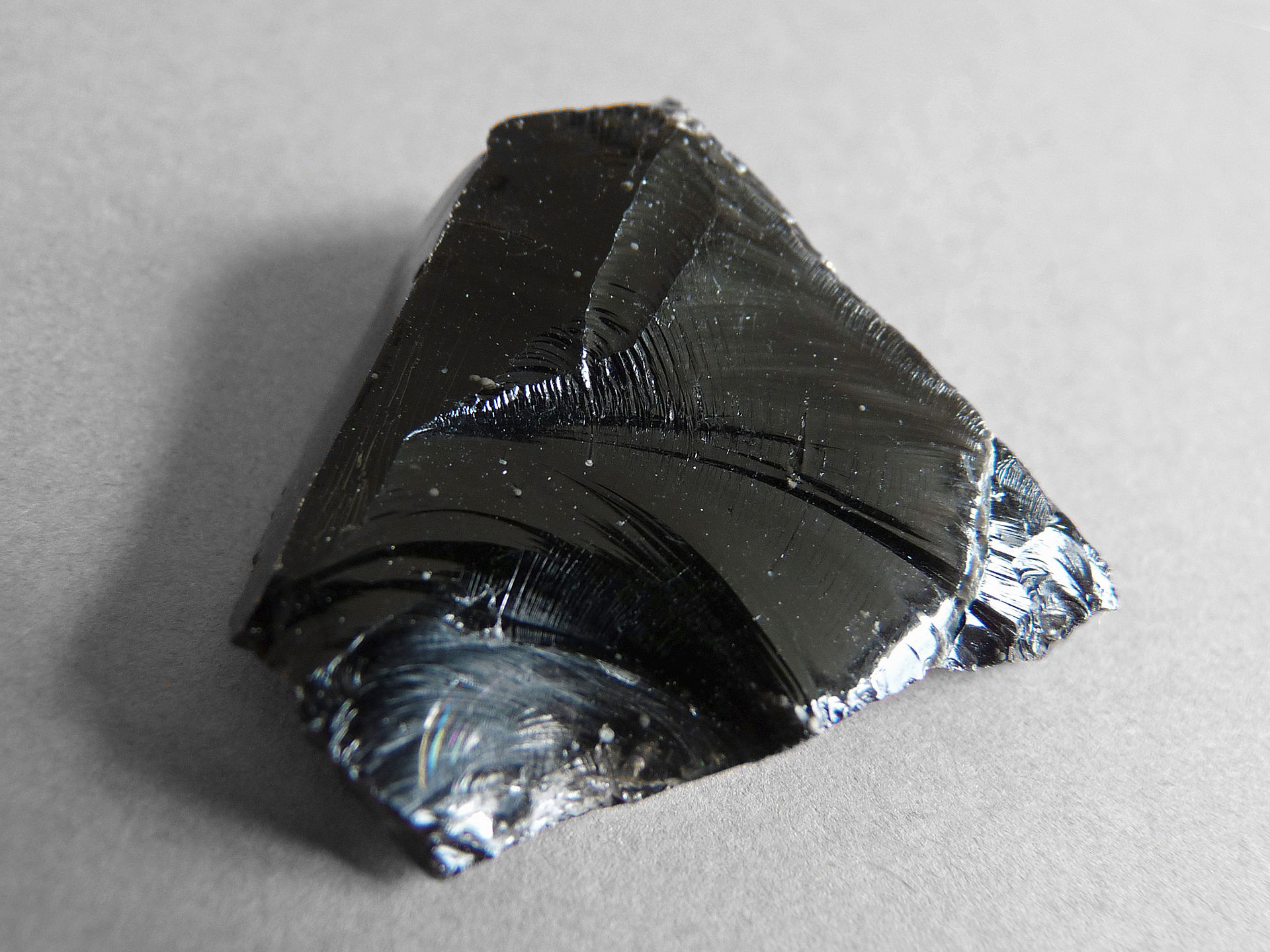
Lava that cools extremely quickly may not form crystals at all, even microscopic ones. The resulting rock is called volcanic glass. Obsidian is a rock consisting of volcanic glass. Obsidian as a glassy rock shows an excellent example of conchoidal fracture similar to the mineral quartz (see chapter 3).
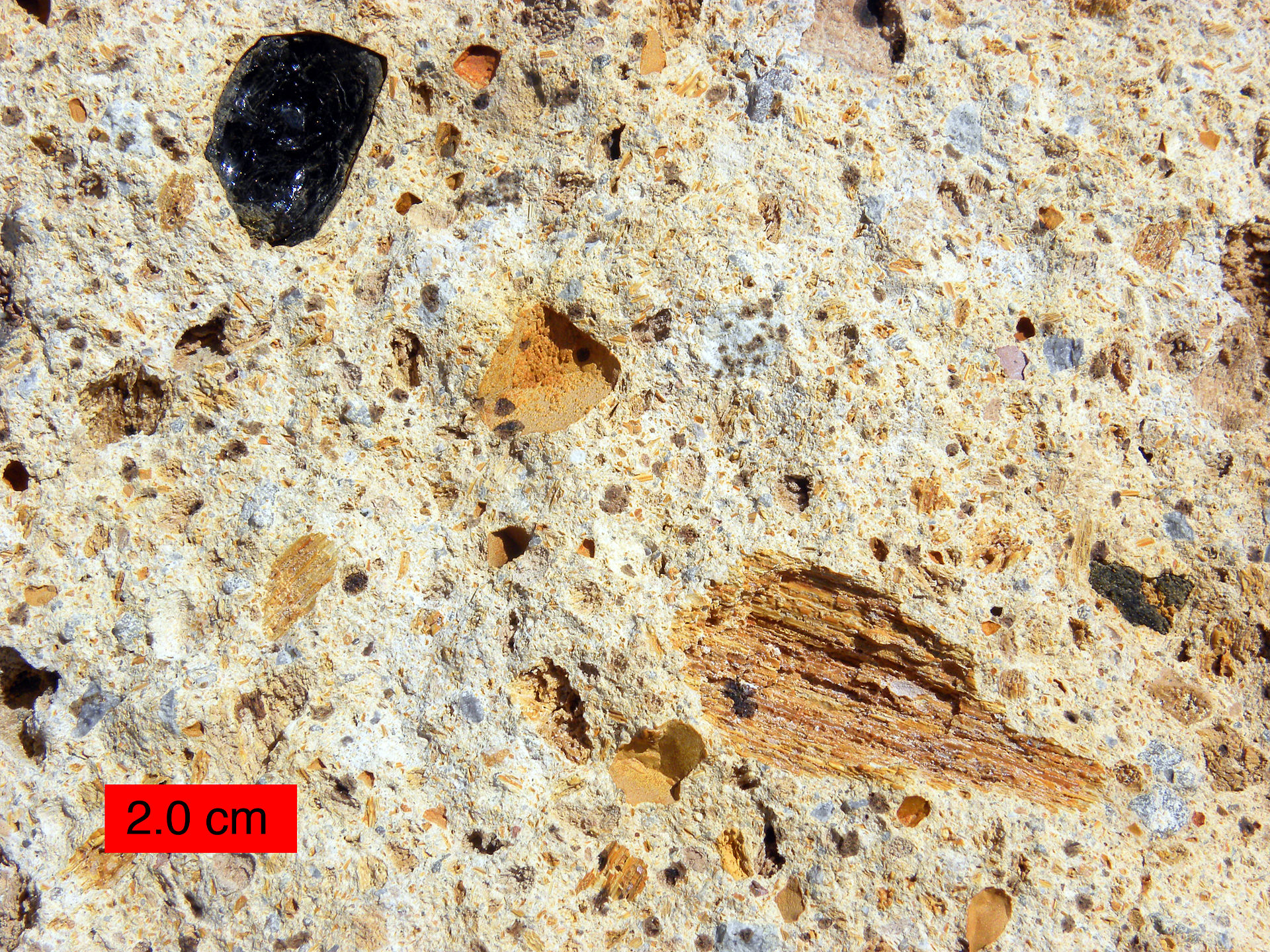
When volcanoes erupt explosively, vast amounts of lava, rock, ash, and gases are thrown into the atmosphere. The solid parts, called tephra, settle back to earth and cool into rocks with pyroclastic textures. Pyro, meaning fire, refers to the igneous source of the tephra and clastic refers to the rock fragments. Tephra fragments are named based on size—ash (<2 mm), lapilli (2-64 mm), and bombs or blocks (>64 mm). Pyroclastic texture is usually recognized by the chaotic mix of crystals, angular glass shards, and rock fragments. Rock formed from large deposits of tephra fragments is called tuff. If the fragments accumulate while still hot, the heat may deform the crystals and weld the mass together, forming a welded tuff.
4.1.2 Composition
Composition refers to a rock’s chemical and mineral make-up . For igneous rock, composition is divided into four groups: felsic, intermediate, mafic, and ultramafic. These groups refer to differing amounts of silica, iron, and magnesium found in the minerals that make up the rocks. It is important to realize these groups do not have sharp boundaries in nature, but rather lie on a continuous spectrum with many transitional compositions and names that refer to specific quantities of minerals. As an example, granite is a commonly-used term, but has a very specific definition which includes exact quantities of minerals like feldspar and quartz. Rocks labeled as ‘granite‘ in laymen applications can be several other rocks, including syenite, tonalite, and monzonite. To avoid these complications, the following figure presents a simplified version of igneous rock nomenclature focusing on the four main groups, which is adequate for an introductory student.
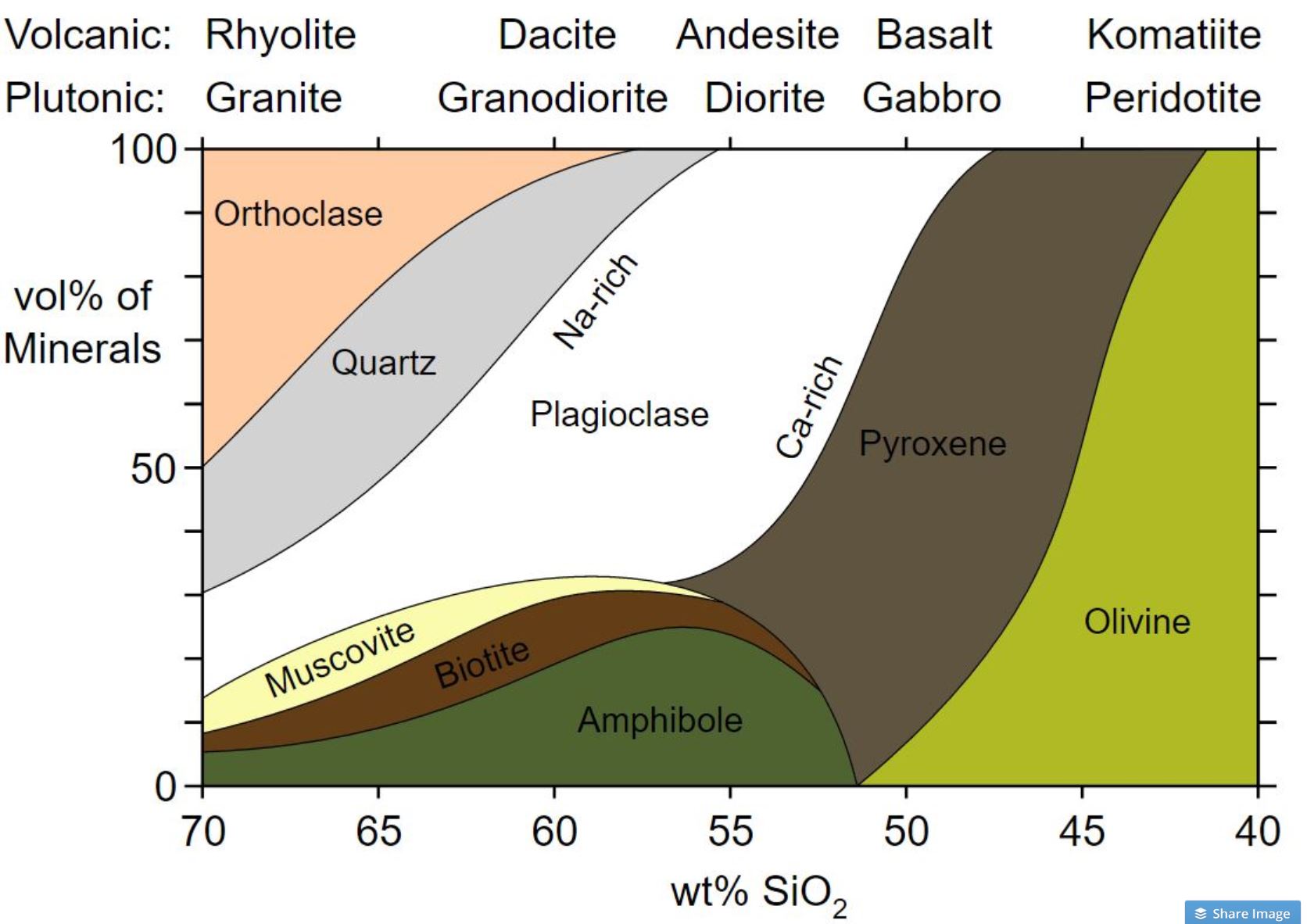
Felsic refers to a predominance of the light-colored (felsic) minerals feldspar and silica in the form of quartz. These light-colored minerals have more silica as a proportion of their overall chemical formula. Minor amounts of dark-colored (mafic) minerals like amphibole and biotite mica may be present as well. Felsic igneous rocks are rich in silica (in the 65-75% range, meaning the rock would be 65-75% weight percent SiO2) and poor in iron and magnesium.
Intermediate is a composition between felsic and mafic. It usually contains roughly-equal amounts of light and dark minerals, including light grains of plagioclase feldspar and dark minerals like amphibole. It is intermediate in silica in the 55-60% range.
Mafic refers to a abundance of ferromagnesian minerals (with magnesium and iron, chemical symbols Mg and Fe) plus plagioclase feldspar. It is mostly made of dark minerals like pyroxene and olivine, which are rich in iron and magnesium and relatively poor in silica. Mafic rocks are low in silica, in the 45-50% range.
Ultramafic refers to the extremely mafic rocks composed of mostly olivine and some pyroxene which have even more magnesium and iron and even less silica. These rocks are rare on the surface, but make up peridotite, the rock of the upper mantle. It is poor in silica, in the 40% or less range.
On the figure above, the top row has both plutonic and volcanic igneous rocks arranged in a continuous spectrum from felsic on the left to intermediate, mafic, and ultramafic toward the right. Rhyolite thus refers to the volcanic and felsic igneous rocks, and granite thus refer to intrusive and felsic igneous rocks. Andesite and diorite likewise refer to extrusive and intrusive intermediate rocks (with dacite and granodiorite applying to those rocks with composition between felsic and intermediate). Basalt and gabbro are the extrusive and intrusive names for mafic igneous rocks, and peridotite is ultramafic, with komatiite as the fine-grained extrusive equivalent. Komatiite is a rare rock because volcanic material that comes direct from the mantle is not common, although some examples can be found in ancient Archean rocks. Nature rarely has sharp boundaries and the classification and naming of rocks often imposes what appear to be sharp boundary names onto a continuous spectrum.
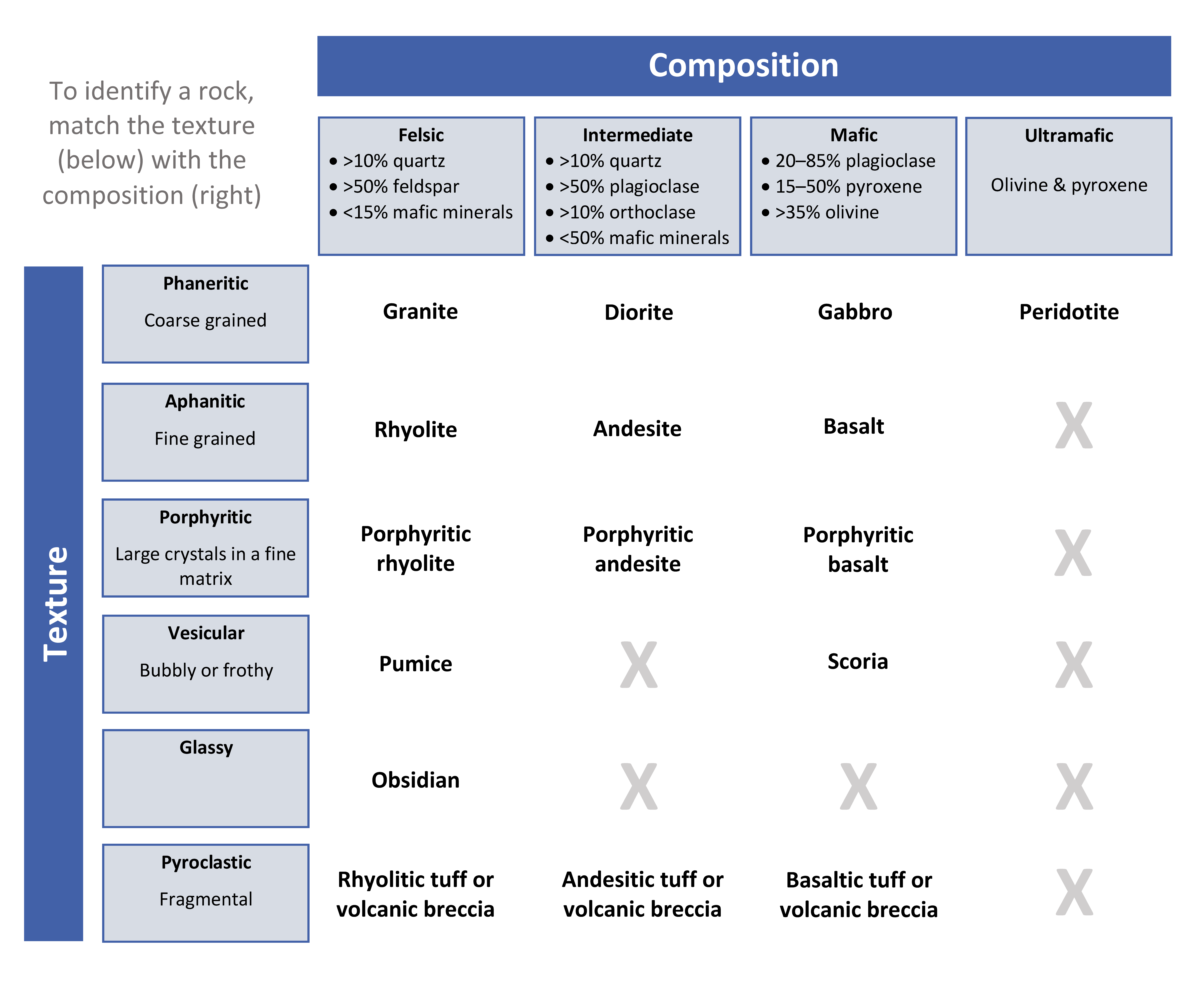
Aphanitic/phaneritic rock types | |
---|---|
Felsic composition | |
![]() |
![]() |
Granite is a course-crystalline felsic intrusive rock. The presence of quartz is a good indicator of granite. Granite commonly has large amounts of salmon pink potassium feldspar and white plagioclase crystals that have visible cleavage planes. Granite is a good approximation for the continental crust, both in density and composition. | Rhyolite is a fine-crystalline felsic extrusive rock. Rhyolite is commonly pink and will often have glassy quartz phenocrysts. Because felsic lavas are less mobile, it is less common than granite. Examples of rhyolite include several lava flows in Yellowstone National Park and the altered rhyolite that makes up the Grand Canyon of the Yellowstone. |
Intermediate composition | |
![]() |
![]() |
Diorite is a coarse-crystalline intermediate intrusive igneous rock. Diorite is identifiable by it’s Dalmatian-like appearance of black hornblende and biotite and white plagioclase feldspar. It is found in its namesake, the Andes Mountains as well as the Henry and Abajo mountains of Utah. | Andesite is a fine crystalline intermediate extrusive rock. It is commonly grey and porphyritic. It can be found in the Andes Mountains and in some island arcs (see Chapter 2). It is the fine grained compositional equivalent of diorite. |
Mafic composition | |
![]() |
![]() |
Gabbro is a coarse-grained mafic igneous rock, made with mainly mafic minerals like pyroxene and only minor plagioclase. Because mafic lava is more mobile, it is less common than basalt. Gabbro is a major component of the lower oceanic crust. | Basalt is a fine-grained mafic igneous rock. It is commonly vesicular and aphanitic. When porphyritic, it often has either olivine or plagioclase phenocrysts. Basalt is the main rock which is formed at mid-ocean ridges, and is therefore the most common rock on the Earth’s surface, making up the entirety of the ocean floor (except where covered by sediment). |
Table 4.1: Aphanitic and phaneritic rock types with images.
4.1.3 Igneous Rock Bodies
Igneous rocks are common in the geologic record, but surprisingly, it is the intrusive rocks that are more common. Extrusive rocks, because of their small crystals and glass, are less durable. Plus, they are, by definition, exposed to the elements of erosion immediately. Intrusive rocks, forming underground with larger, stronger crystals, are more likely to last. Therefore, most landforms and rock groups that owe their origin to igneous rocks are intrusive bodies. A significant exception to this is active volcanoes, which are discussed in a later section on volcanism. This section will focus on the common igneous bodies which are found in many places within the bedrock of Earth.
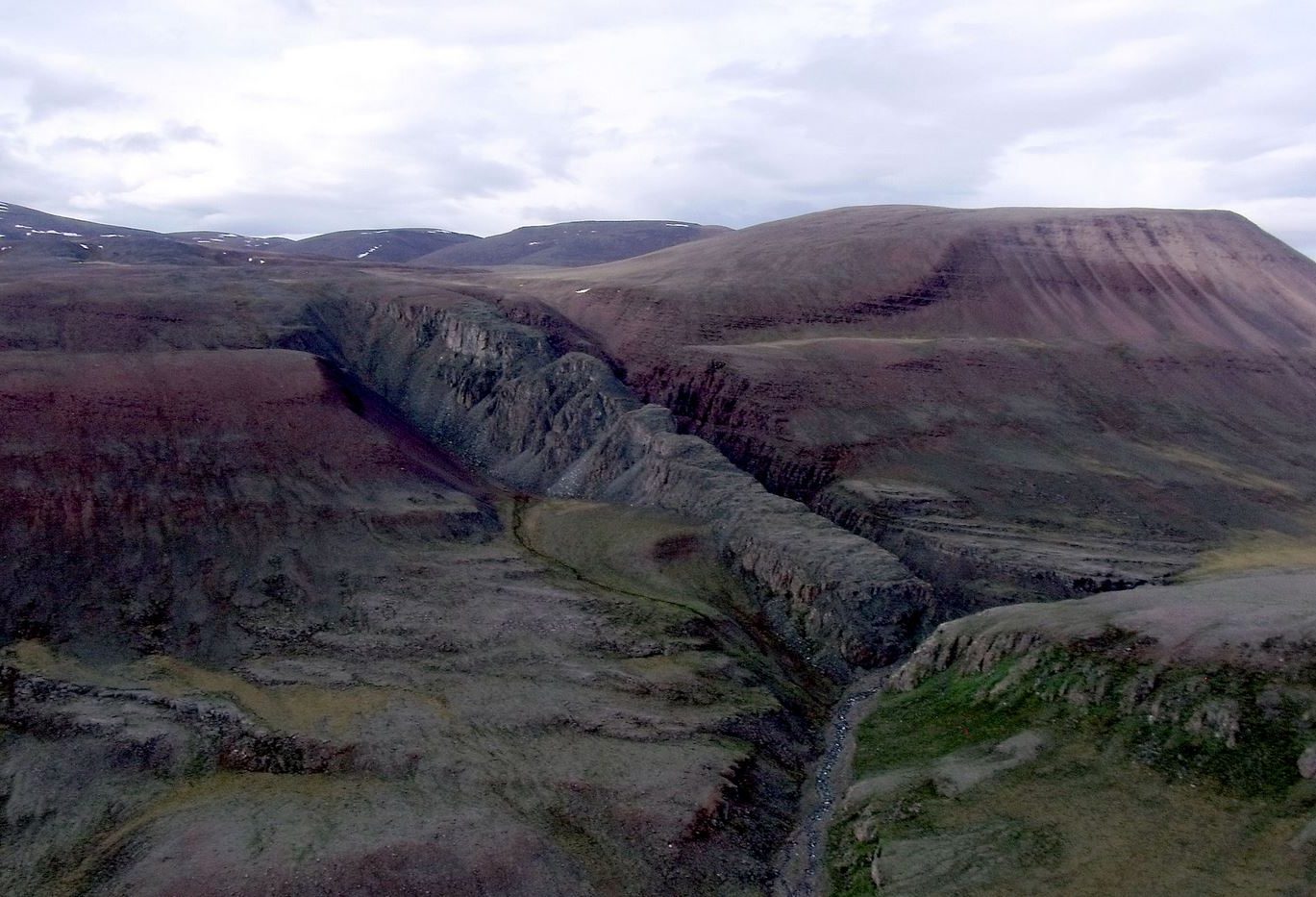
When magma intrudes into a weakness like a crack or fissure and solidifies, the resulting cross-cutting feature is called a dike (sometimes spelled dyke). Because of this, dikes are often vertical or at an angle relative to the pre-existing rock layers that they intersect. Dikes are therefore discordant intrusions, not following any layering that was present. Dikes are important to geologists, not only for the study of igneous rocks themselves but also for dating rock sequences and interpreting the geologic history of an area. The dike is younger than the rocks it cuts across and, as discussed in the chapter on Geologic Time (chapter 7), may be used to assign actual numeric ages to sedimentary sequences, which are notoriously difficult to age date.
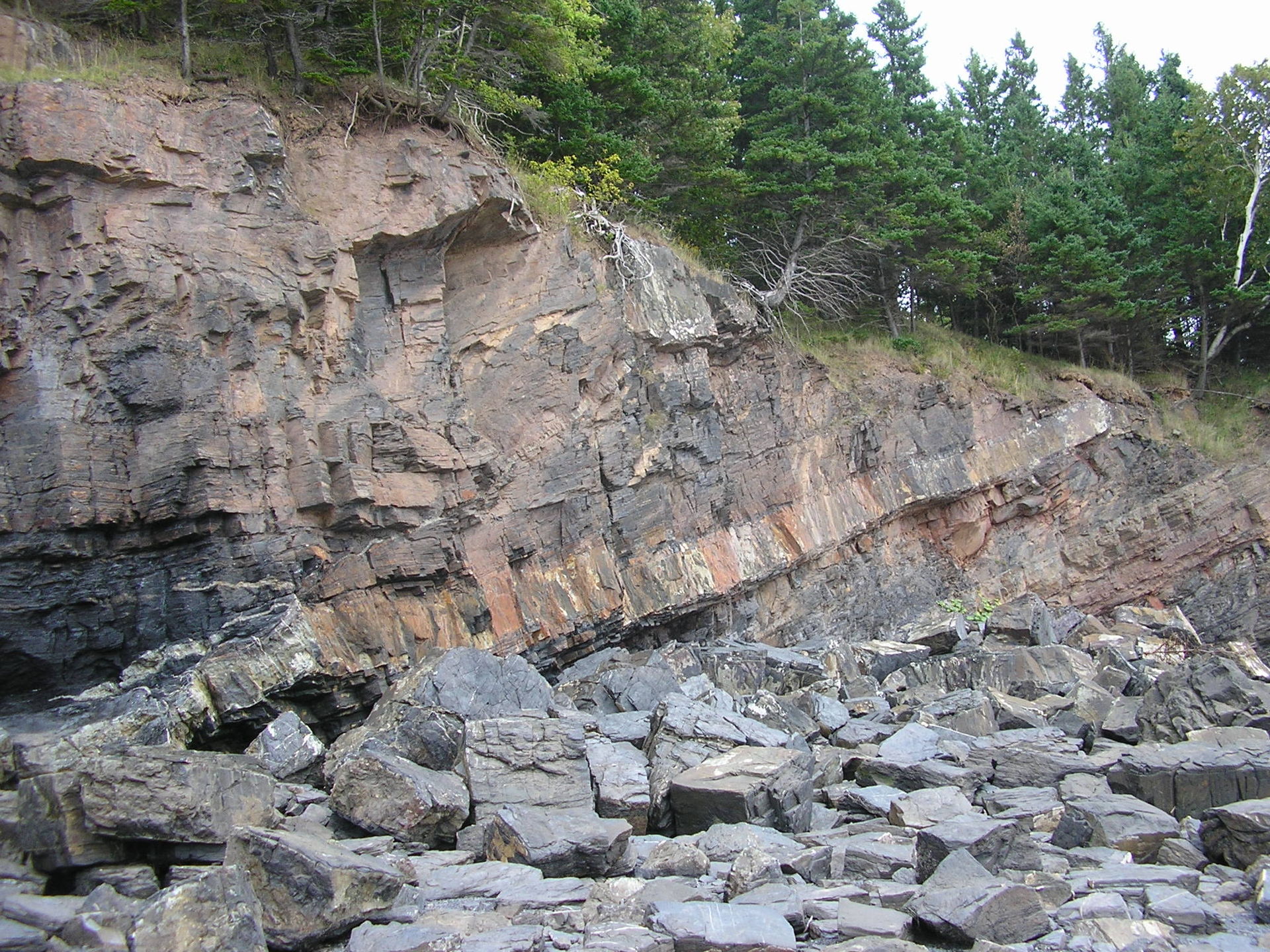
Sills are another type of intrusive structure. A sill is a concordant intrusion that runs parallel to the sedimentary layers in the country rock. They are formed when magma exploits a weakness between these layers, shouldering them apart and squeezing between them. As with dikes, sills are younger than the surrounding layers and may be radioactively dated to study the age of sedimentary strata.
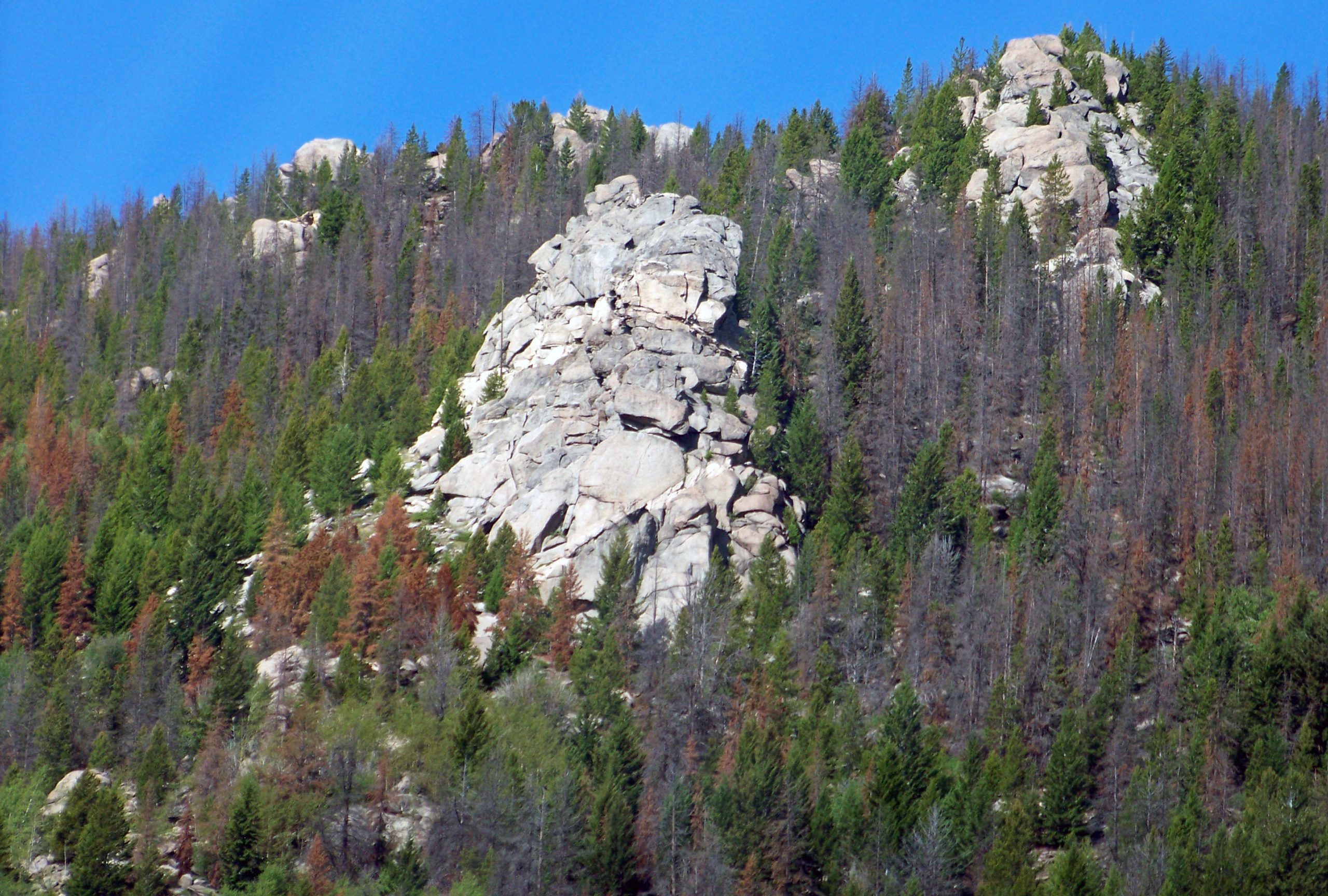
A magma chamber is a large underground reservoir of molten rock. The path of rising magma is called a diapir. The processes by which a diapir intrudes into the surrounding native or country rock are not well understood and are the subject of ongoing geological inquiry. For example, it is not known what happens to the pre-existing country rock as the diapir intrudes. One theory is the overriding rock gets shouldered aside, displaced by the increased volume of magma. Another is the native rock is melted and consumed into the rising magma or broken into pieces that settle into the magma, a process known as stoping. It has also been proposed that diapirs are not a real phenomenon, but just a series of dikes that blend into each other. The dikes may be intruding over millions of years, but since they may be made of similar material, they would be appearing to be formed at the same time. Regardless, when a diapir cools, it forms an mass of intrusive rock called a pluton. Plutons can have irregular shapes, but can often be somewhat round.
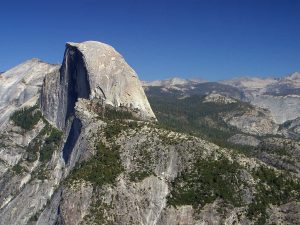
When many plutons merge together in an extensive single feature, it is called a batholith. Batholiths are found in the cores of many mountain ranges, including the granite formations of Yosemite National Park in the Sierra Nevada of California. They are typically more than 100 km2 in area, associated with subduction zones, and mostly felsic in composition. A stock is a type of pluton with less surface exposure than a batholith, and may represent a narrower neck of material emerging from the top of a batholith. Batholiths and stocks are discordant intrusions that cut across and through surrounding country rock.
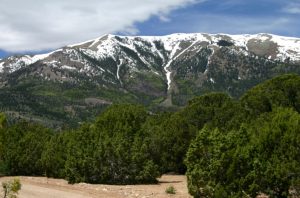
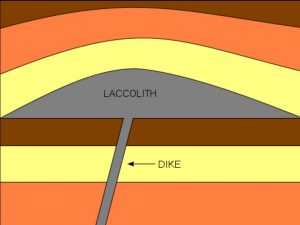
Laccoliths are blister-like, concordant intrusions of magma that form between sedimentary layers. The Henry Mountains of Utah are a famous topographic landform formed by this process. Laccoliths bulge upwards; a similar downward-bulging intrusion is called a lopolith.
Complete this interactive activity to check your understanding. Click on the plus signs on the illustration for descriptions of several igneous features.
If you are using an offline version of this text, access this interactive activity via the QR code.
Take this quiz to check your comprehension of this section.
If you are using an offline version of this text, access the quiz for section 4.1 via the QR code.
4.2 Bowen’s Reaction Series
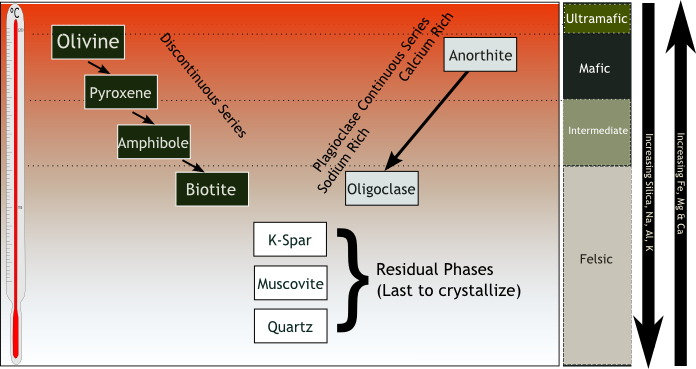
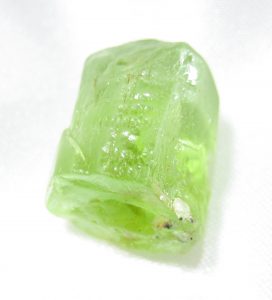
Bowen’s Reaction Series describes the temperature at which minerals crystallize when cooled, or melt when heated. The low end of the temperature scale where all minerals crystallize into solid rock, is approximately 700°C (1292°F). The upper end of the range where all minerals exist in a molten state, is approximately 1,250°C (2,282°F). These numbers reference minerals that crystallize at standard sea-level pressure, 1 bar. The values will be different for minerals located deep below the Earth’s surface due to the increased pressure, which affects crystallization and melting temperatures. However, the order and relationships are maintained.
In the figure, the righthand column lists the four groups of igneous rock from top to bottom: ultramafic, mafic, intermediate, and felsic. The down-pointing arrow on the far right shows increasing amounts of silica, sodium, aluminum, and potassium as the mineral composition goes from ultramafic to felsic. The up-pointing arrow shows increasing ferromagnesian components, specifically iron, magnesium, and calcium. To the far left of the diagram is a temperature scale. Minerals near the top of diagram, such as olivine and anorthite (a type of plagioclase), crystallize at higher temperatures. Minerals near the bottom, such as quartz and muscovite, crystalize at lower temperatures.
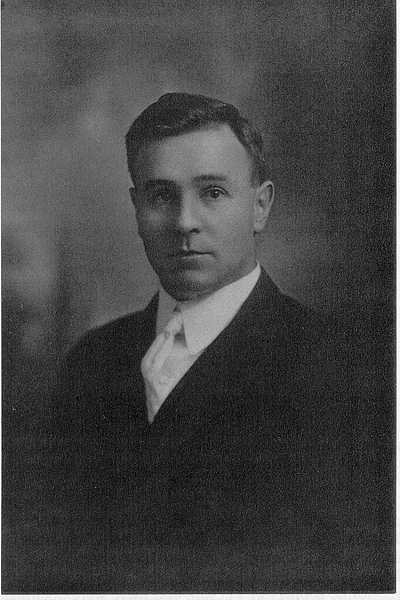
The most important aspect of Bowen's Reaction Series is to notice the relationships between minerals and temperature. Norman L. Bowen (1887-1956) was an early 20th Century geologist who studied igneous rocks. He noticed that in igneous rocks, certain minerals always occur together and these mineral assemblages exclude other minerals. Curious as to why, and with the hypothesis in mind that it had to do with the temperature at which the rocks cooled, he set about conducting experiments on igneous rocks in the early 1900s. He conducted experiments on igneous rock—grinding combinations of rocks into powder, sealing the powders into metal capsules, heating them to various temperatures, and then cooling them.
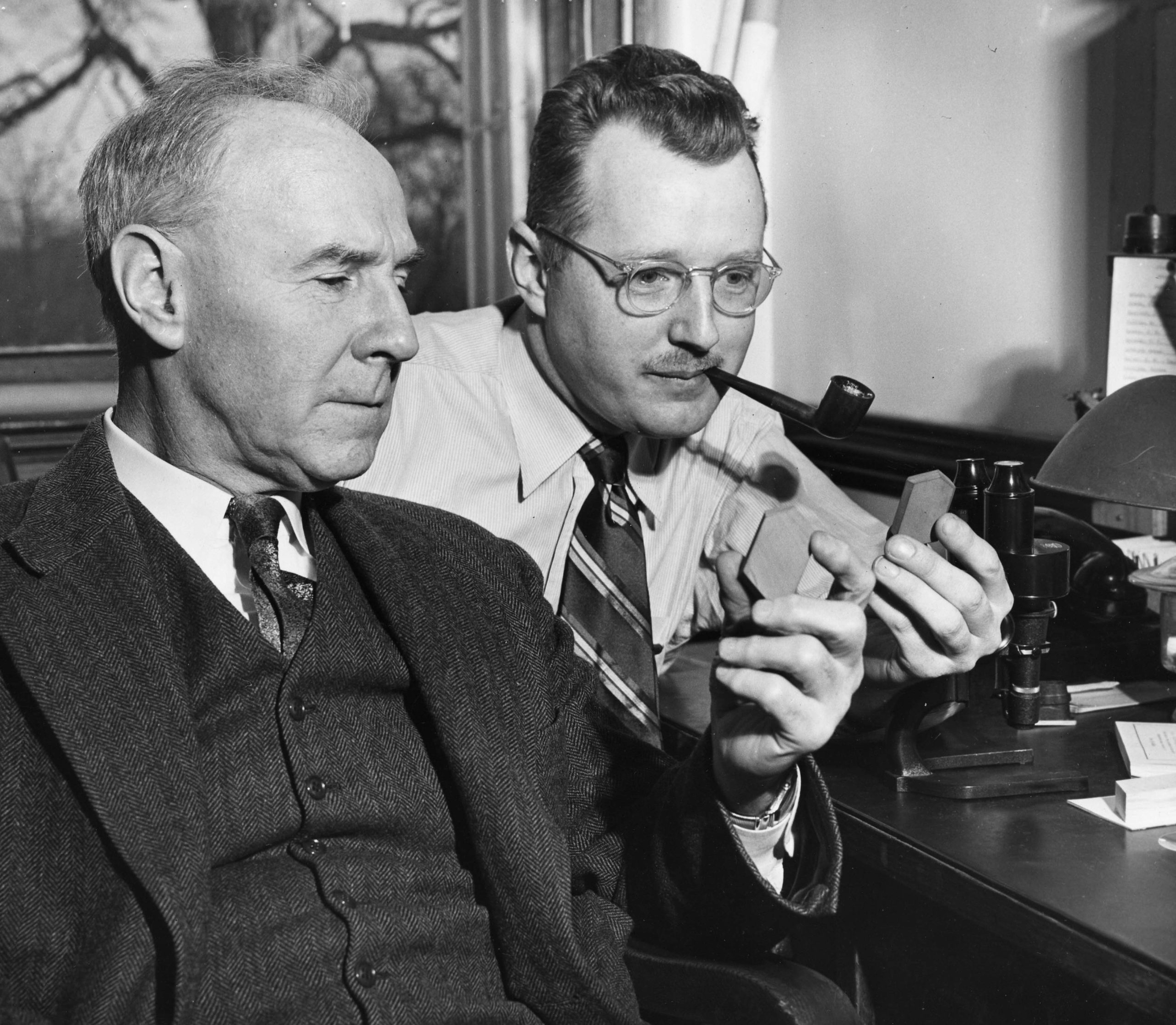
When he opened the quenched capsules, he found a glass surrounding mineral crystals that he could identify under his petrographic microscope. The results of many of these experiments, conducted at different temperatures over a period of several years, showed that the common igneous minerals crystallize from magma at different temperatures. He also saw that minerals occur together in rocks with others that crystallize within similar temperature ranges, and never crystallize with other minerals. This relationship can explain the main difference between mafic and felsic igneous rocks. Mafic igneous rocks contain more mafic minerals, and therefore, crystallize at higher temperatures than felsic igneous rocks. This is even seen in lava flows, with felsic lavas erupting hundreds of degrees cooler than their mafic counterparts. Bowen’s work laid the foundation for understanding igneous petrology (the study of rocks) and resulted in his book, The Evolution of the Igneous Rocks in 1928.
Take this quiz to check your comprehension of this section.
If you are using an offline version of this text, access the quiz for section 4.2 via the QR code.
4.3 Magma Generation
Magma and lava contain three components: melt, solids, and volatiles. The melt is made of ions from minerals that have liquefied. The solids are made of crystallized minerals floating in the liquid melt. These may be minerals that have already cooled Volatiles are gaseous components—such as water vapor, carbon dioxide, sulfur, and chlorine—dissolved in the magma. The presence and amount of these three components affect the physical behavior of the magma and will be discussed more below.
4.3.1 Geothermal Gradient
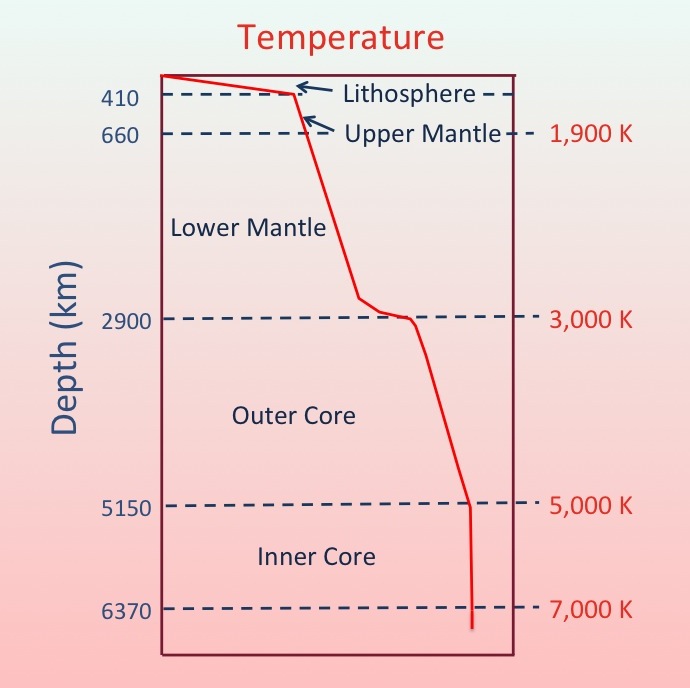
Below the surface, the temperature of the Earth rises. This heat is caused by residual heat left from the formation of Earth and ongoing radioactive decay. The rate at which temperature increases with depth is called the geothermal gradient. The average geothermal gradient in the upper 100 km (62 mi) of the crust is about 25°C per kilometer of depth. So for every kilometer of depth, the temperature increases by about 25°C.
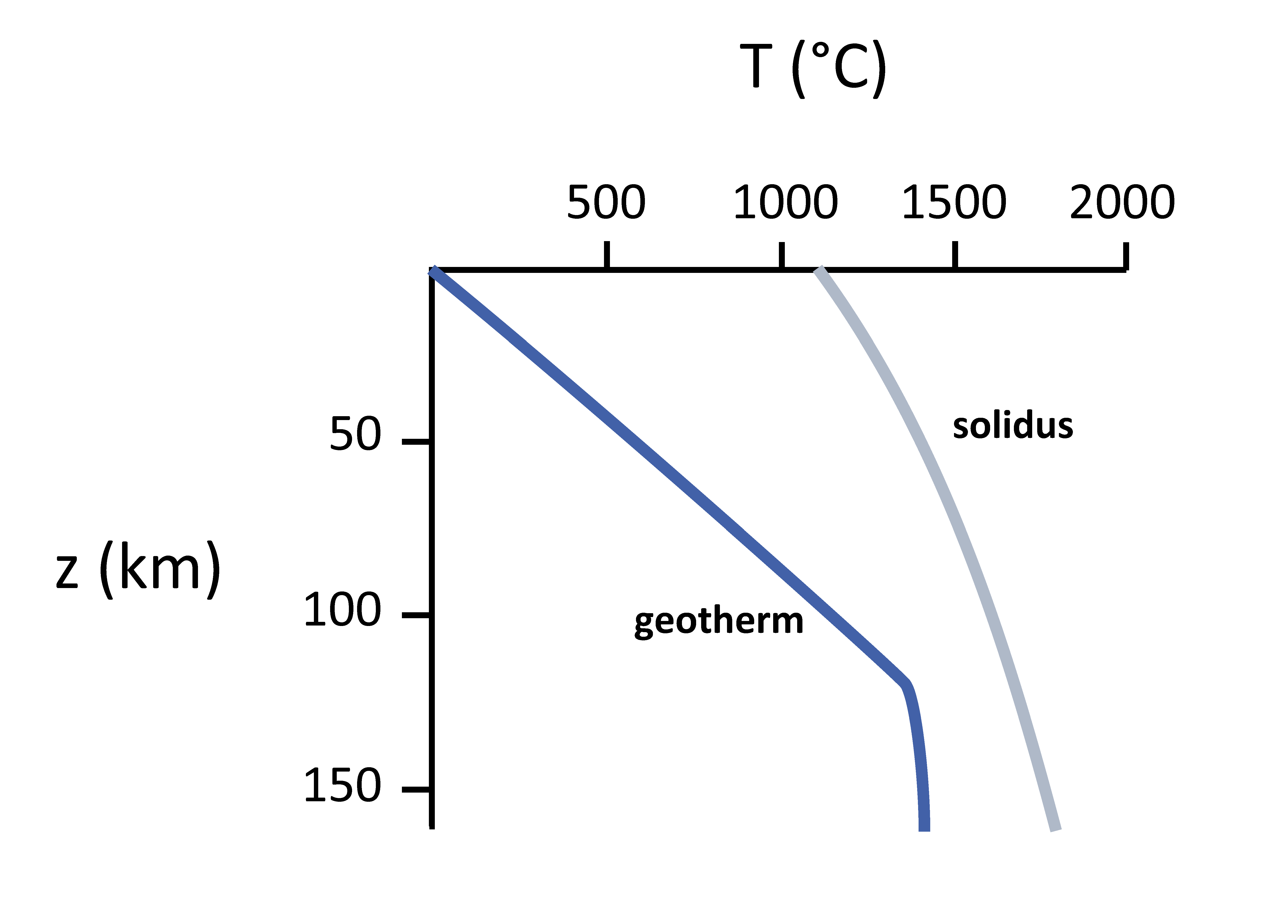
The depth-temperature graph (see figure 4.23) illustrates the relationship between the geothermal gradient (geotherm, red line) and the start of rock melting (solidus, green line). The geothermal gradient changes with depth (which has a direct relationship to pressure) through the crust into upper mantle. The area to the left of the green line includes solid components; to the right is where liquid components start to form. The increasing temperature with depth makes the depth of about 125 kilometers (78 miles) where the natural geothermal gradient is closest to the solidus.
The temperature at 100 km (62 mi) deep is about 1,200°C (2,192°F). At bottom of the crust, 35 km (22 mi) deep, the pressure is about 10,000 bars. A bar is a measure of pressure, with 1 bar being normal atmospheric pressure at sea level. At these pressures and temperatures, the crust and mantle are solid. To a depth of 150 km (93 mi), the geothermal gradient line stays to the left of the solidus line. This relationship continues through the mantle to the core–mantle boundary, at 2,880 km (1,790 mi).
The solidus line slopes to the right because the melting temperature of any substance depends on pressure. The higher pressure created at greater depth increases the temperature needed to melt rock. In another example, at sea level with an atmospheric pressure close to 1 bar, water boils at 100°C. But if the pressure is lowered, as shown on the video below, water boils at a much lower temperature.
Video 4.1: Boiling water at room temperature.
If you are using an offline version of this text, access this YouTube video via the QR code.
There are three principal ways rock behavior crosses to the right of the green solidus line to create molten magma: 1) decompression melting caused by lowering the pressure, 2) flux melting caused by adding volatiles (see more below), and 3) heat-induced melting caused by increasing the temperature. The Bowen’s Reaction Series shows that minerals melt at different temperatures. Since magma is a mixture of different minerals, the solidus boundary is more of a fuzzy zone rather than a well-defined line; some minerals are melted and some remain solid. This type of rock behavior is called partial melting and represents real-world magmas, which typically contain solid, liquid, and volatile components.
4.4 Volcanism
When magma emerges onto the Earth’s surface, the molten rock is called lava. A volcano is a type of land formation created when lava solidifies into rock. Volcanoes have been an important part of human society for centuries, though their understanding has greatly increased as our understanding of plate tectonics has made them less mysterious. This section describes volcano location, type, hazards, and monitoring.
4.4.1. Distribution and Tectonics
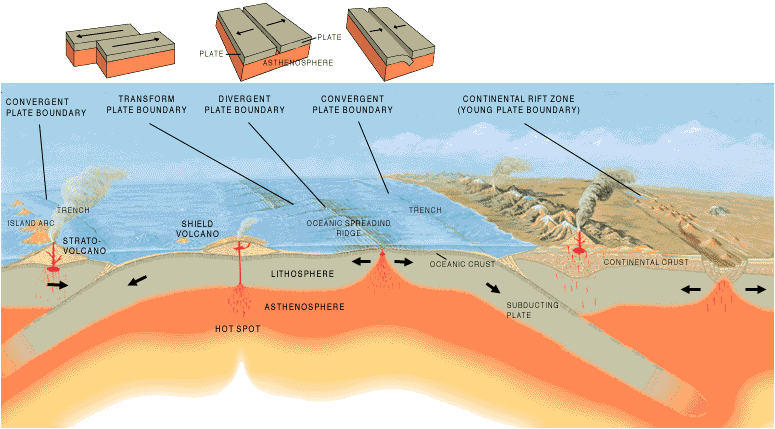
Most volcanoes are interplate volcanoes. Interplate volcanoes are located at active plate boundaries created by volcanism at mid-ocean ridges, subduction zones, and continental rifts. The prefix “inter-“ means between. Some volcanoes are intraplate volcanoes. The prefix “intra-“ means within, and intraplate volcanoes are located within tectonic plates, far removed from plate boundaries. Many intraplate volcanoes are formed by hotspots.
Volcanoes at Mid-ocean Ridges
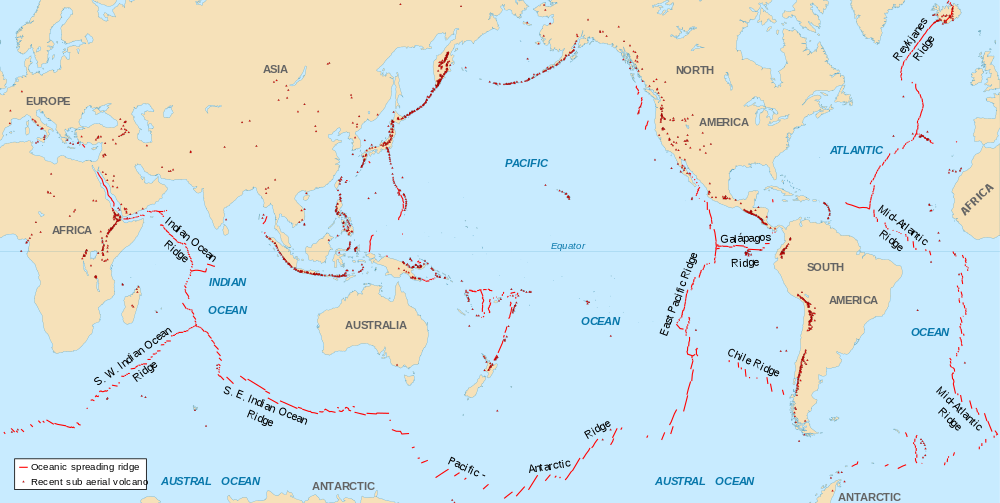
Most volcanism on Earth occurs on the ocean floor along mid-ocean ridges, a type of divergent plate boundary (see chapter 2). These interplate volcanoes are also the least observed and famous, since most of them are located under 3,000-4,500 m (10,000-15,000 ft) of ocean and the eruptions are slow, gentle, and oozing. One exception is the interplate volcanoes of Iceland. The diverging and thinning oceanic plates allow hot mantle rock to rise, releasing pressure and causing decompression melting. Ultramafic mantle rock, consisting largely of peridotite, partially melts and generates magma that is basaltic. Because of this, almost all volcanoes on the ocean floor are basaltic. In fact, most oceanic lithosphere is basaltic near the surface, with phaneritic gabbro and ultramafic peridotite underneath.
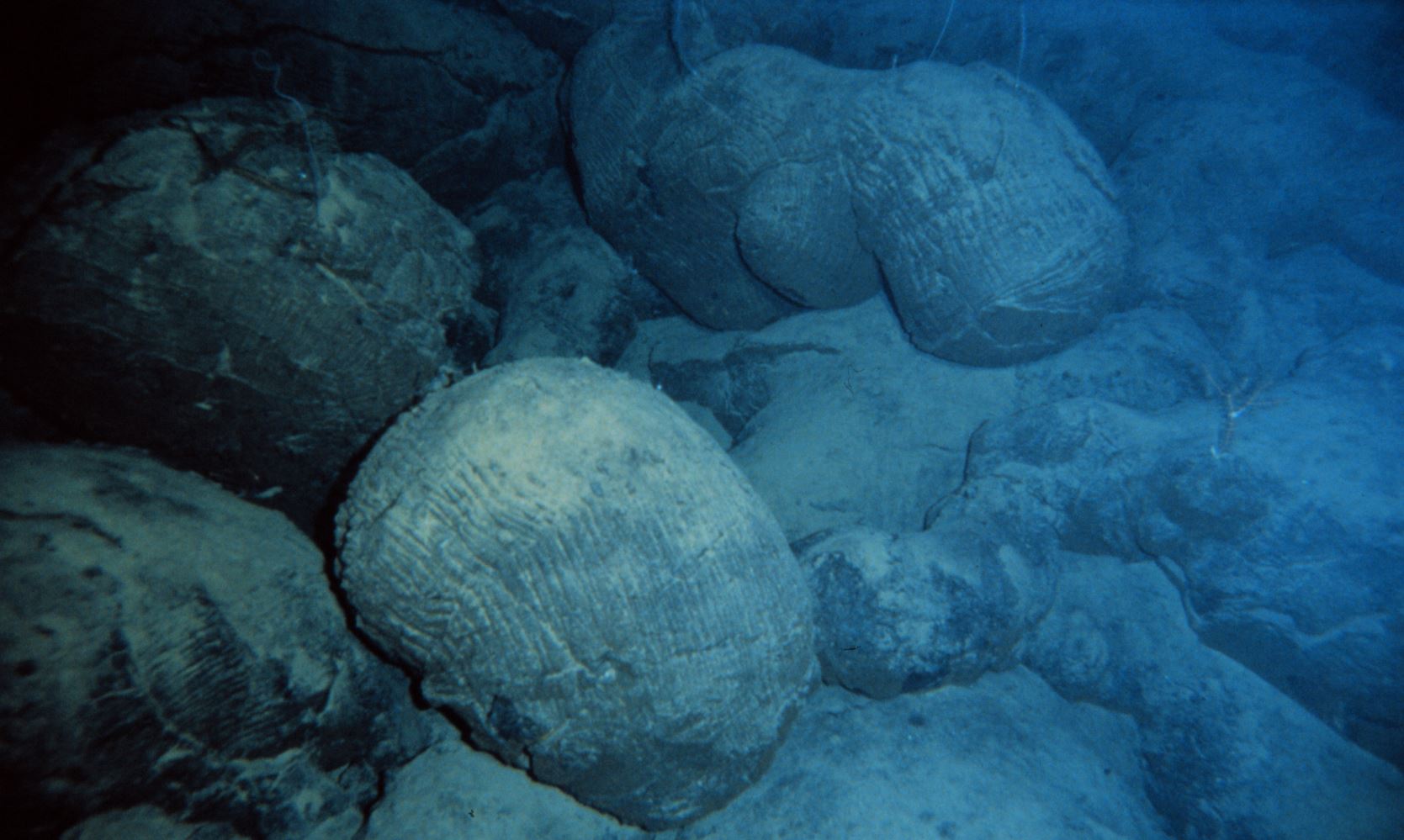
When basaltic lava erupts underwater it emerges in small explosions and/or forms pillow-shaped structures called pillow basalts. These seafloor eruptions enable entire underwater ecosystems to thrive in the deep ocean around mid-ocean ridges. This ecosystem exists around tall vents emitting black, hot mineral-rich water called deep-sea hydrothermal vents, also known as black smokers.
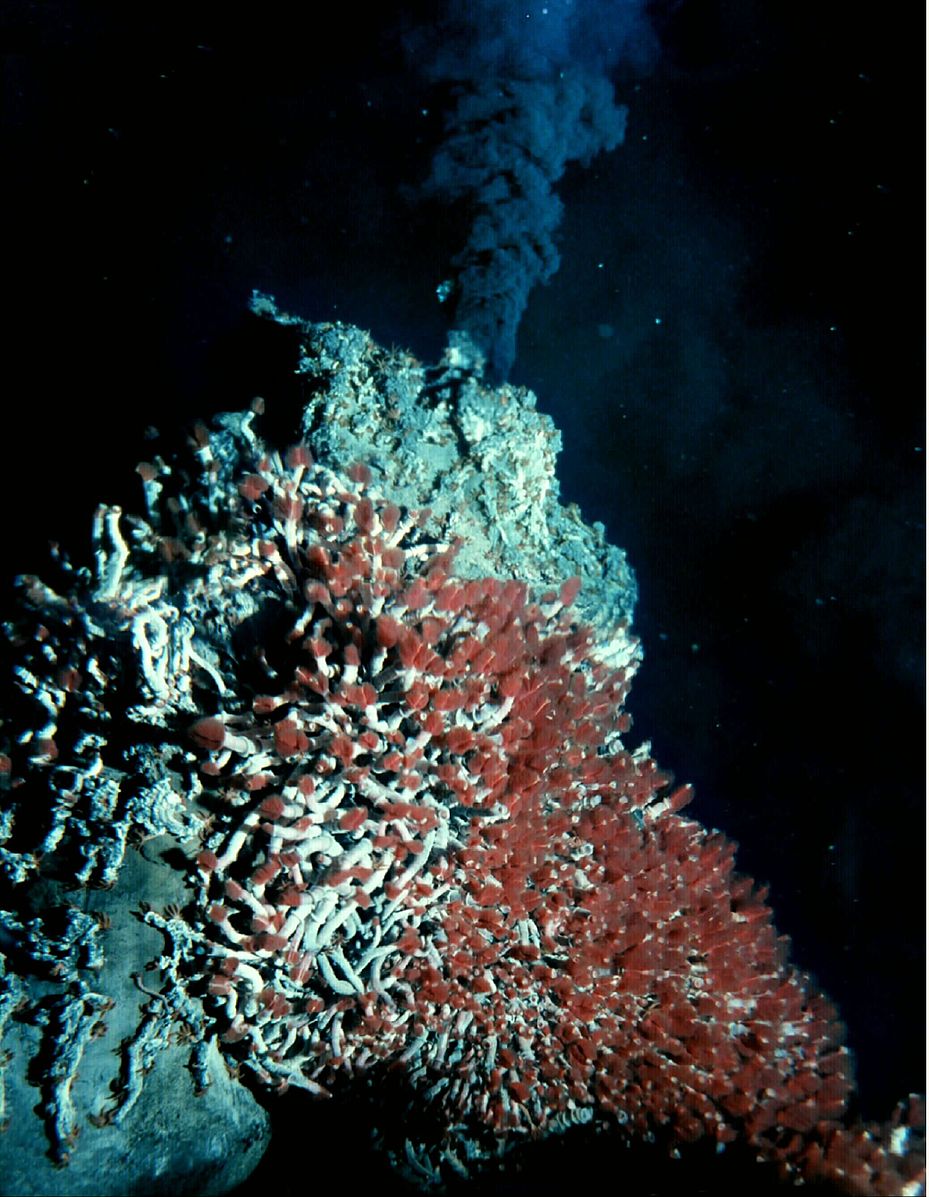
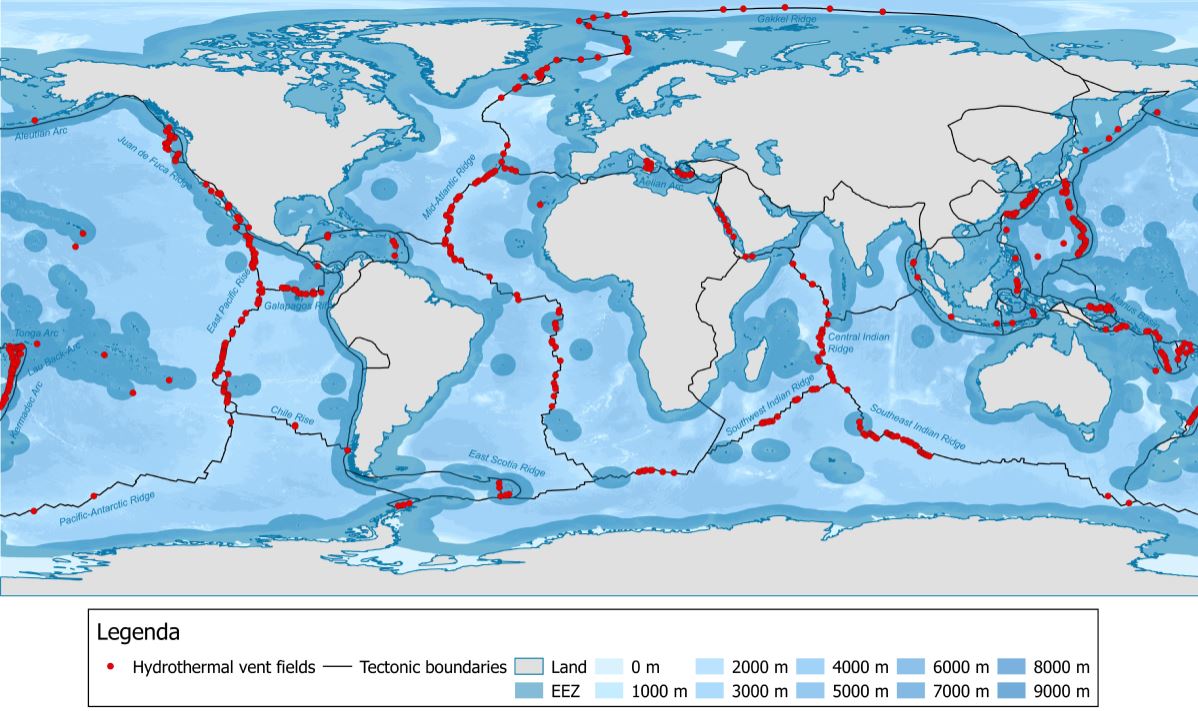
Without sunlight to support photosynthesis, these organisms instead utilize a process called chemosynthesis. Certain bacteria are able to turn hydrogen sulfide (H2S), a gas that smells like rotten eggs, into life-supporting nutrients and water. Larger organisms may eat these bacteria or absorb nutrients and water produced by bacteria living symbiotically inside their bodies. The videos show some of the ecosystems found around deep-sea hydrothermal vents.
Video 4.2: Updating the deep–diving submarine at 50-years-old.
If you are using an offline version of this text, access this YouTube video via the QR code.
Video 4.3: Incredible views on board the deep-sea vessel.
If you are using an offline version of this text, access this YouTube video via the QR code.
Volcanoes at Subduction Zones
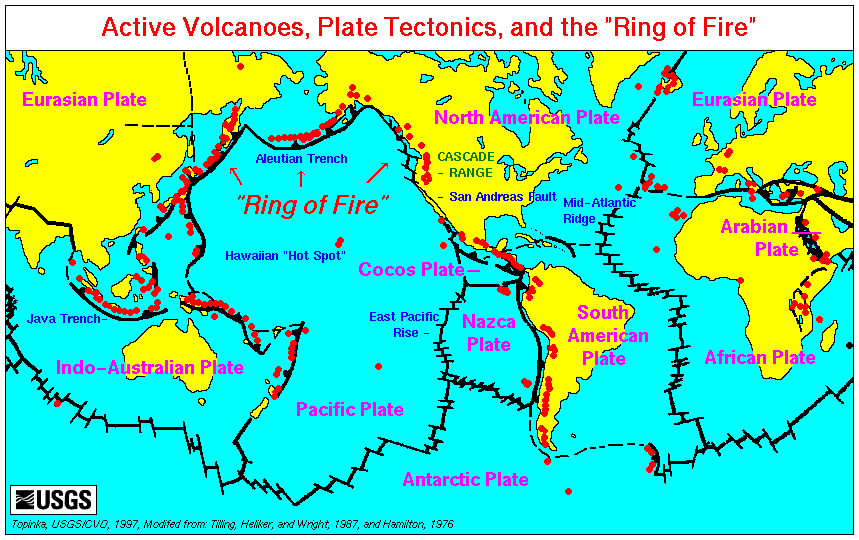
The second most commonly found location for volcanism is adjacent to subduction zones, a type of convergent plate boundary (see chapter 2). The process of subduction expels water from hydrated minerals in the descending slab, which causes flux melting in the overlying mantle rock. Because subduction volcanism occurs in a volcanic arc, the thickened crust promotes partial melting and magma differentiation. These evolve the mafic magma from the mantle into more silica-rich magma. The Ring of Fire surrounding the Pacific Ocean, for example, is dominated by subduction-generated eruptions of mostly silica-rich lava; the volcanoes and plutons consist largely of intermediate-to-felsic rock such as andesite, rhyolite, pumice, and tuff.
Volcanoes at Continental Rifts
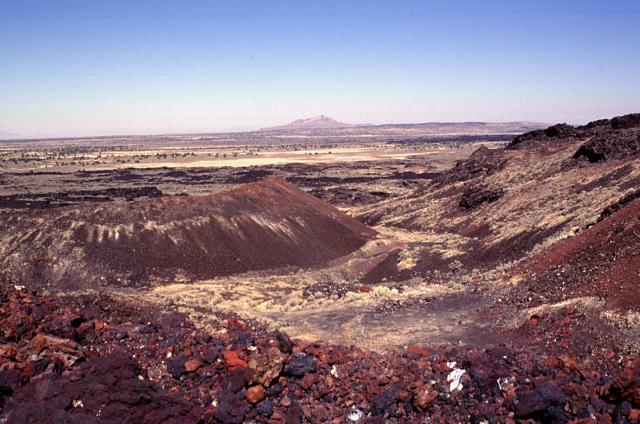
Some volcanoes are created at continental rifts, where crustal thinning is caused by diverging lithospheric plates, such as the East African Rift Basin in Africa. Volcanism caused by crustal thinning without continental rifting is found in the Basin and Range Province in North America. In this location, volcanic activity is produced by rising magma that stretches the overlying crust. Lower crust or upper mantle material rises through the thinned crust, releases pressure, and undergoes decompression-induced partial melting. This magma is less dense than the surrounding rock and continues to rise through the crust to the surface, erupting as basaltic lava. These eruptions usually result in flood basalts, cinder cones, and basaltic lava flows (see video). Relatively young cinder cones of basaltic lava can be found in south-central Utah, in the Black Rock Desert Volcanic Field, which is part of the zone of Basin and Range crustal extension. These Utah cinder cones and lava flows started erupting around 6 million years ago, with the last eruption occurring 720 years ago.
Video 4.4: Basin and range volcanic processes.
If you are using an offline version of this text, access this YouTube video via the QR code.
Hotspots
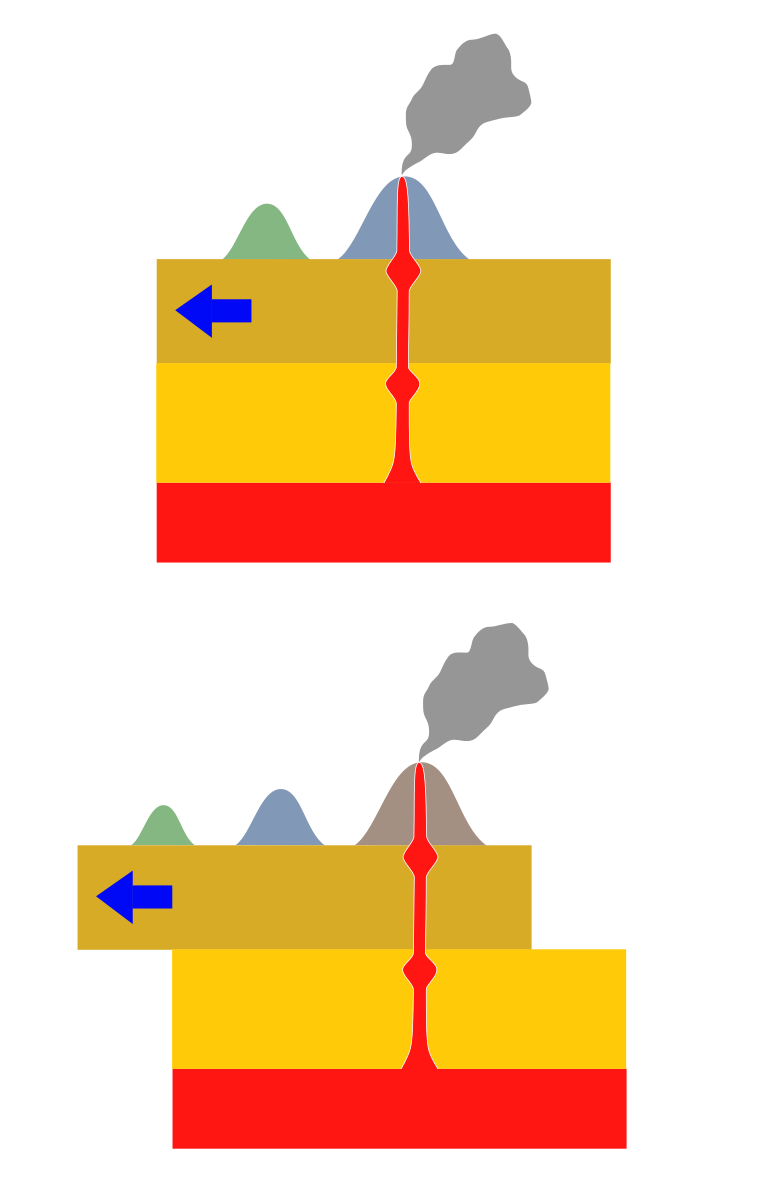
Hotspots are the main source of intraplate volcanism. Hotspots occur when lithospheric plates glide over a hot mantle plume, an ascending column of solid heated rock originating from deep within the mantle. The mantle plume generates melts as material rises, with the magma rising even more. When the ascending magma reaches the lithospheric crust, it spreads out into a mushroom-shaped head that is tens to hundreds of kilometers across.
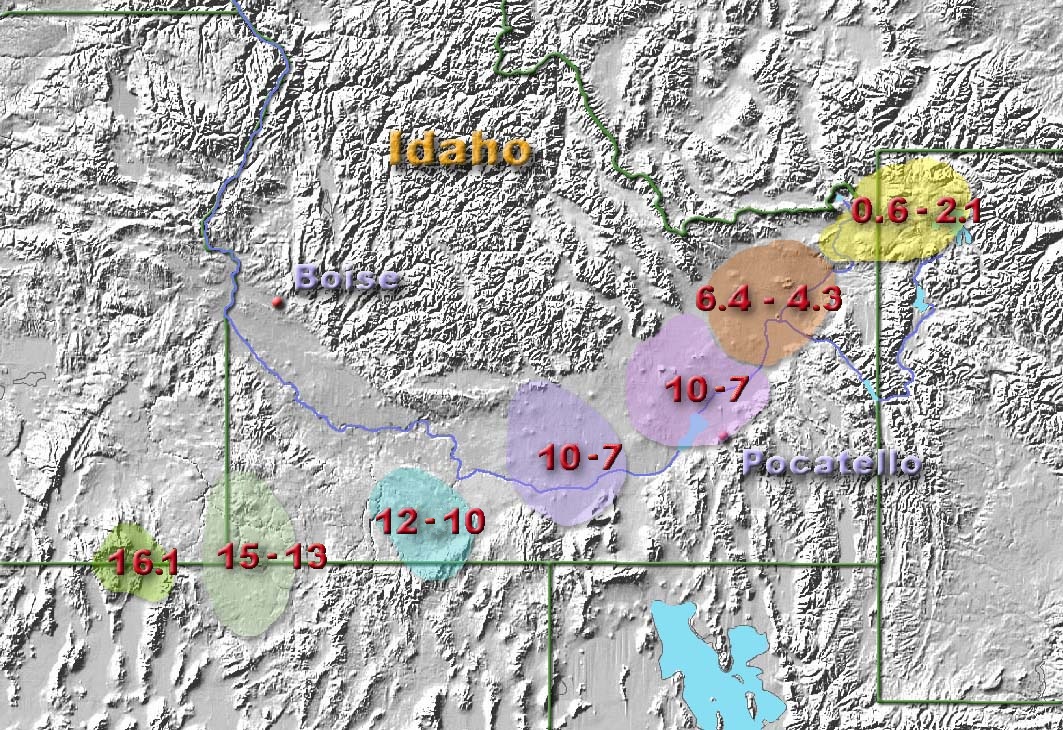
Since most mantle plumes are located beneath the oceanic lithosphere, the early stages of intraplate volcanism typically take place underwater. Over time, basaltic volcanoes may build up from the sea floor into islands, such as the Hawaiian Islands. Where a hotspot is found under a continental plate, contact with the hot mafic magma may cause the overlying felsic rock to melt and mix with the mafic material below, forming intermediate magma. Or the felsic magma may continue to rise, and cool into a granitic batholith or erupt as a felsic volcano. The Yellowstone caldera is an example of hotspot volcanism that resulted in an explosive eruption.
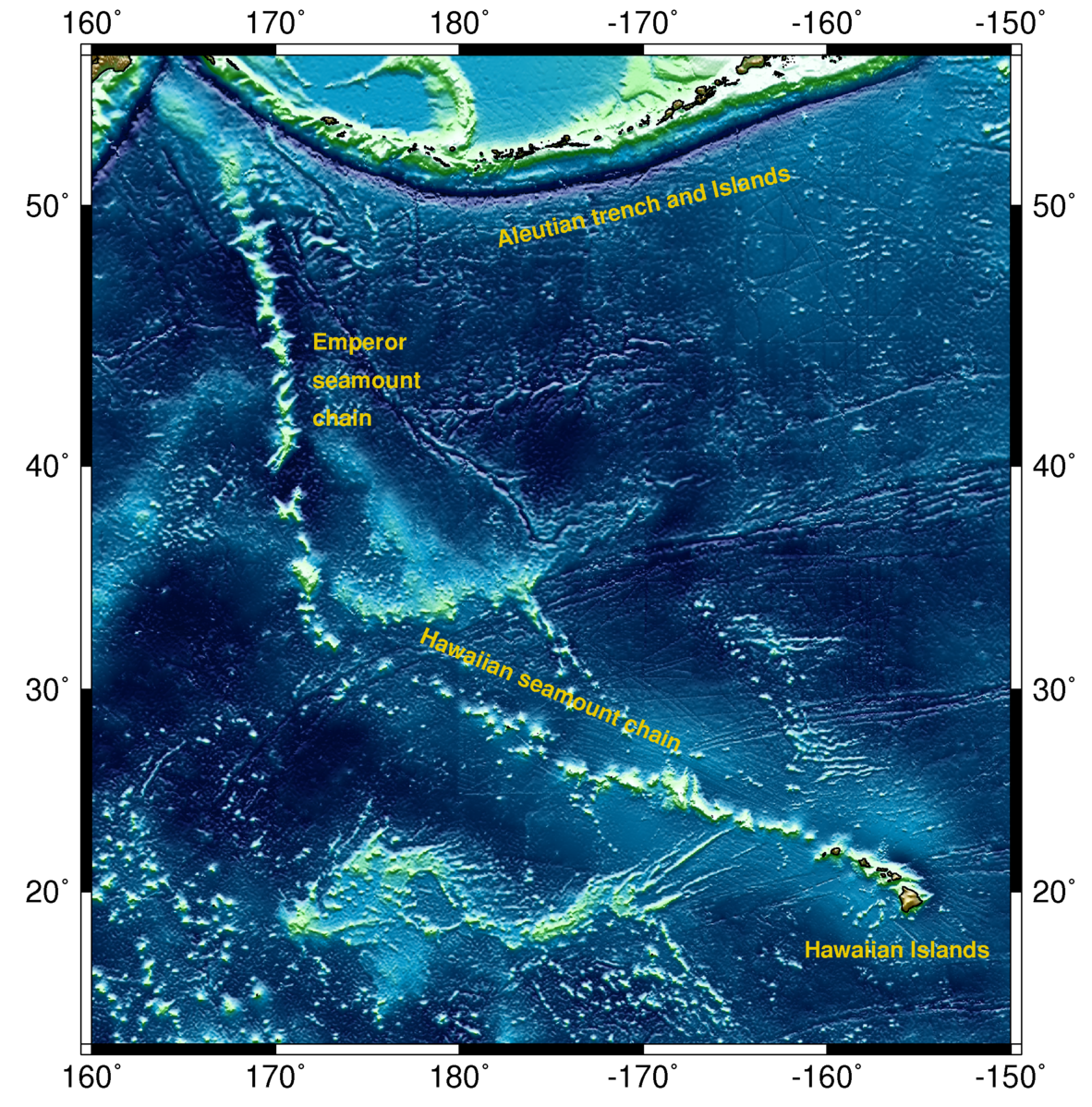
A zone of actively erupting volcanism connected to a chain of extinct volcanoes indicates intraplate volcanism located over a hotspot. These volcanic chains are created by the overriding oceanic plate slowly moving over a hotspot mantle plume. These chains are seen on the seafloor and continents and include volcanoes that have been inactive for millions of years. The Hawaiian Islands on the Pacific Oceanic plate are the active end of a long volcanic chain that extends from the northwest Pacific Ocean to the Emperor Seamounts, all the way to the to the subduction zone beneath the Kamchatka Peninsula. The overriding North American continental plate moved across a mantle plume hotspot for several million years, creating a chain of volcanic calderas that extends from Southwestern Idaho to the presently active Yellowstone caldera in Wyoming.
The three-minute video (below) illustrates hotspot volcanoes.
Video 4.5: What is a volcanic hotspot?
If you are using an offline version of this text, access this YouTube video via the QR code.
4.4.2 Volcano Features and Types
There are several different types of volcanoes based on their shape, eruption style, magmatic composition, and other aspects.
Complete this interactive activity to check your understanding.
If you are using an offline version of this text, access this interactive activity via the QR code.
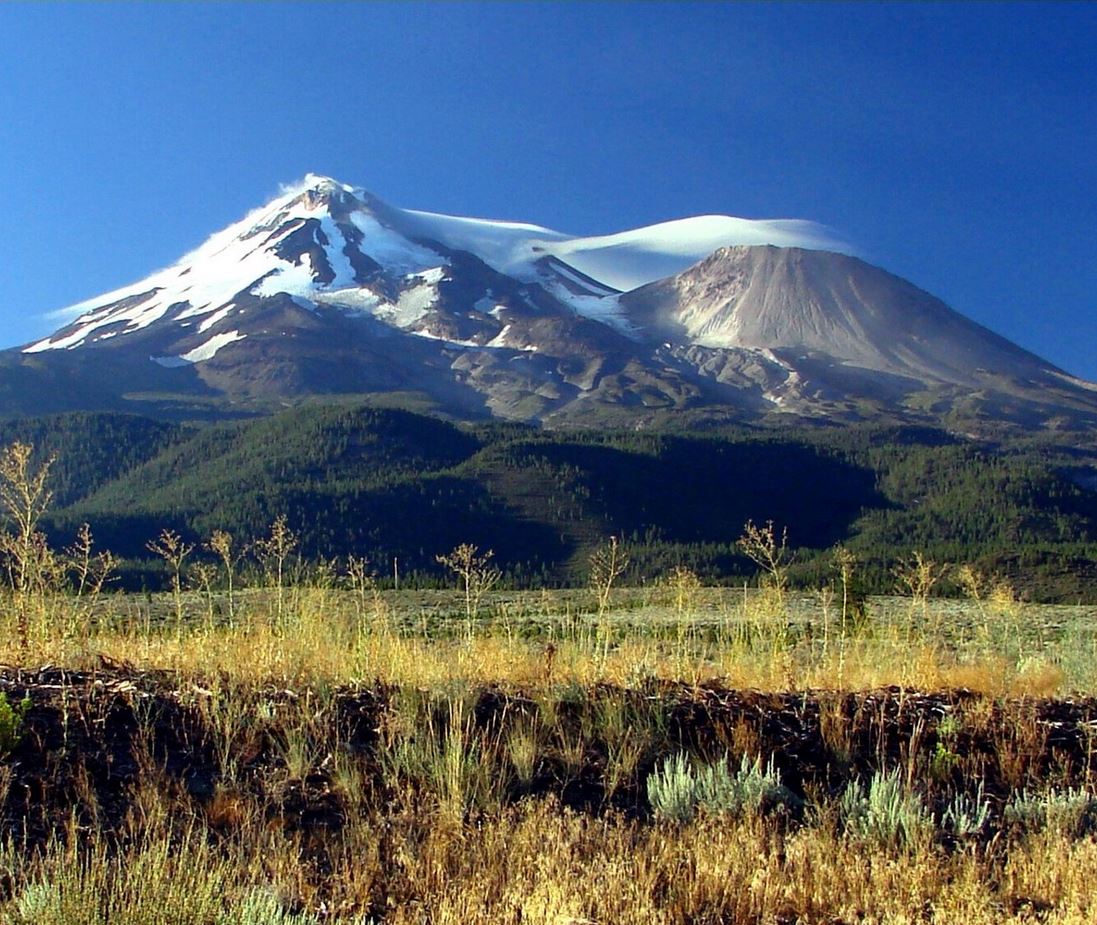
The figure shows the main features of a typical stratovolcano: 1) magma chamber, 2) upper layers of lithosphere, 3) the conduit or narrow pipe through which the lava erupts, 4) the base or edge of the volcano, 5) a sill of magma between layers of the volcano, 6) a diapir or feeder tube to the sill, 7) layers of tephra (ash) from previous eruptions, 8 & 9) layers of lava erupting from the vent and flowing down the sides of the volcano, 10) the crater at the top of the volcano, 11) layers of lava and tephra on (12), a parasitic cone. A parasitic cone is a small volcano located on the flank of a larger volcano such as Shastina on Mount Shasta. Kilauea sitting on the flank of Mauna Loa is not considered a parasitic cone because it has its own separate magma chamber, 13) the vents of the parasite and the main volcano, 14) the rim of the crater, 15) clouds of ash blown into the sky by the eruption; this settles back onto the volcano and surrounding land.
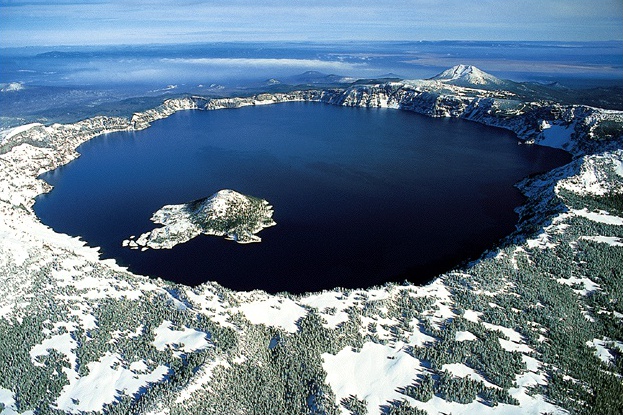
The largest craters are called calderas, such as the Crater Lake Caldera in Oregon. Many volcanic features are produced by viscosity, a basic property of a lava. Viscosity is the resistance to flowing by a fluid. Low viscosity magma flows easily more like syrup, the basaltic volcanism that occurs in Hawai’i on shield volcanoes. High viscosity means a thick and sticky magma, typically felsic or intermediate, that flows slowly, similar to toothpaste.
Shield Volcano
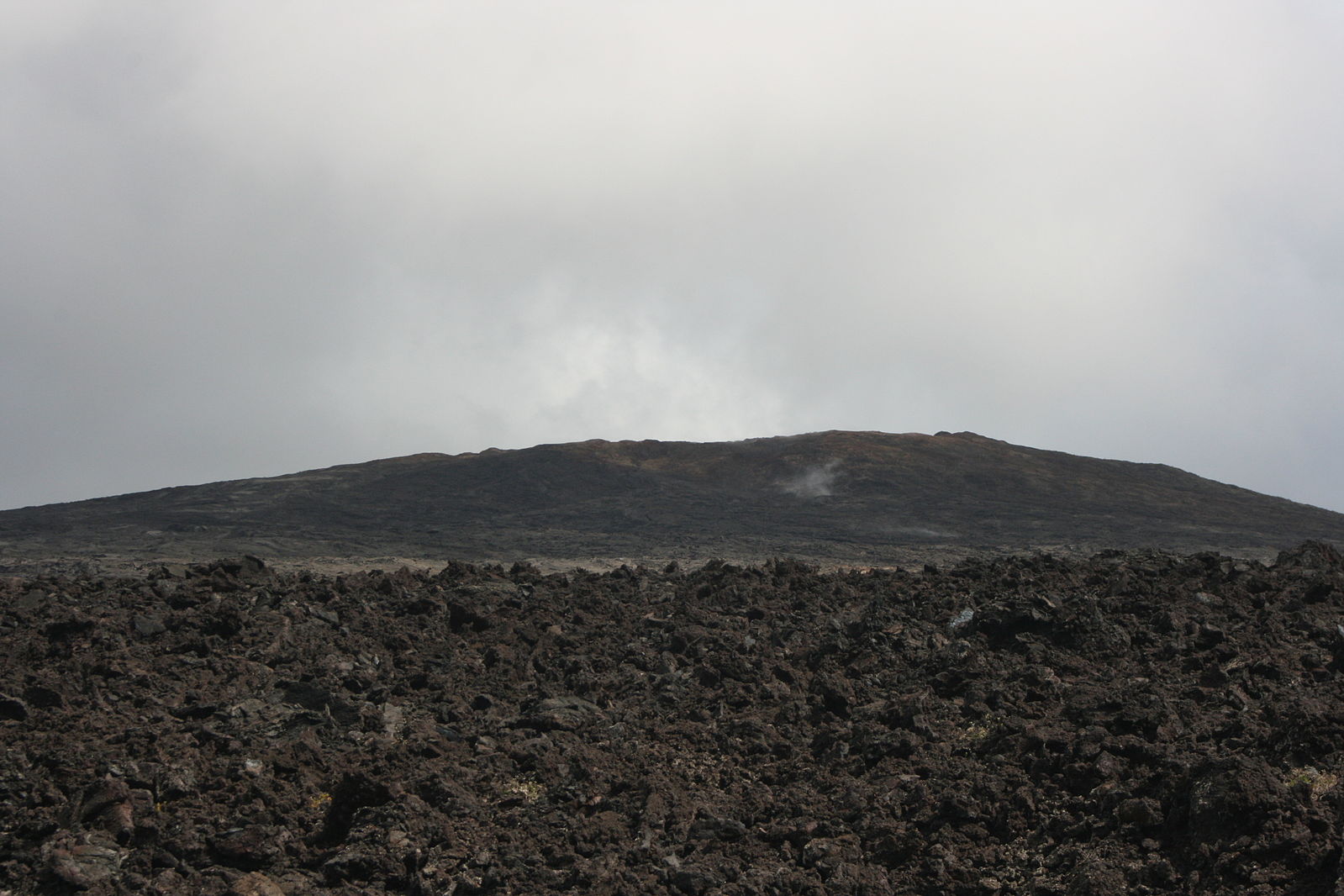
The largest volcanoes are shield volcanoes. They are characterized by broad low-angle flanks, small vents at the top, and mafic magma chambers. The name comes from the side view, which resembles a medieval warrior’s shield. They are typically associated with hotspots, mid-ocean ridges, or continental rifts with rising upper mantle material. The low-angle flanks are built up slowly from numerous low-viscosity basaltic lava flows that spread out over long distances. The basaltic lava erupts effusively, meaning the eruptions are small, localized, and predictable.
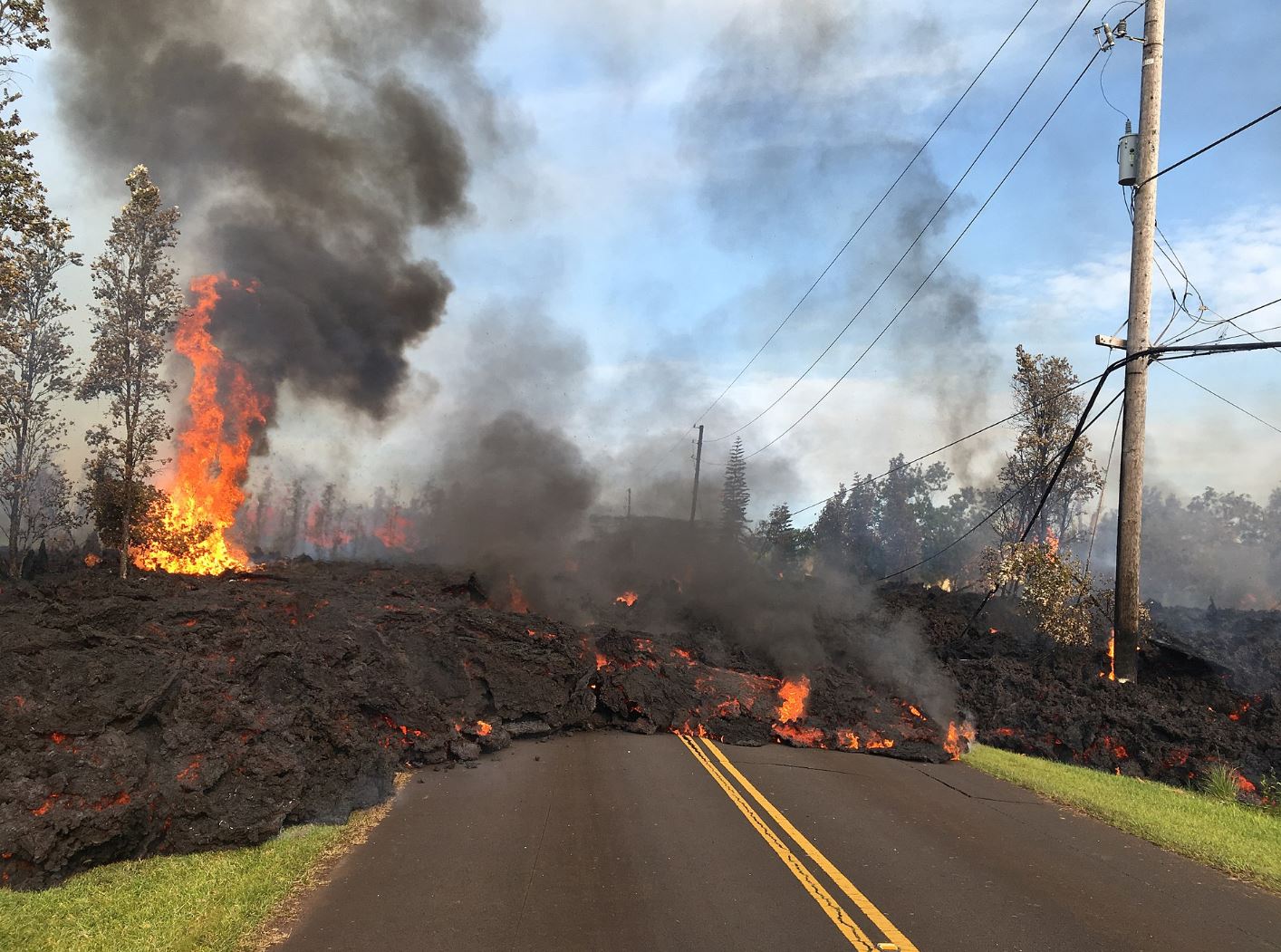
Typically, shield volcano eruptions are not much of a hazard to human life—although non-explosive eruptions of Kilauea (Hawai’i) in 2018 produced uncharacteristically large lavas that damaged roads and structures. Mauna Loa (see USGS page) and Kilauea (see USGS page) in Hawai’i are examples of shield volcanoes. Shield volcanoes are also found in Iceland, the Galapagos Islands, Northern California, Oregon, and the East African Rift.
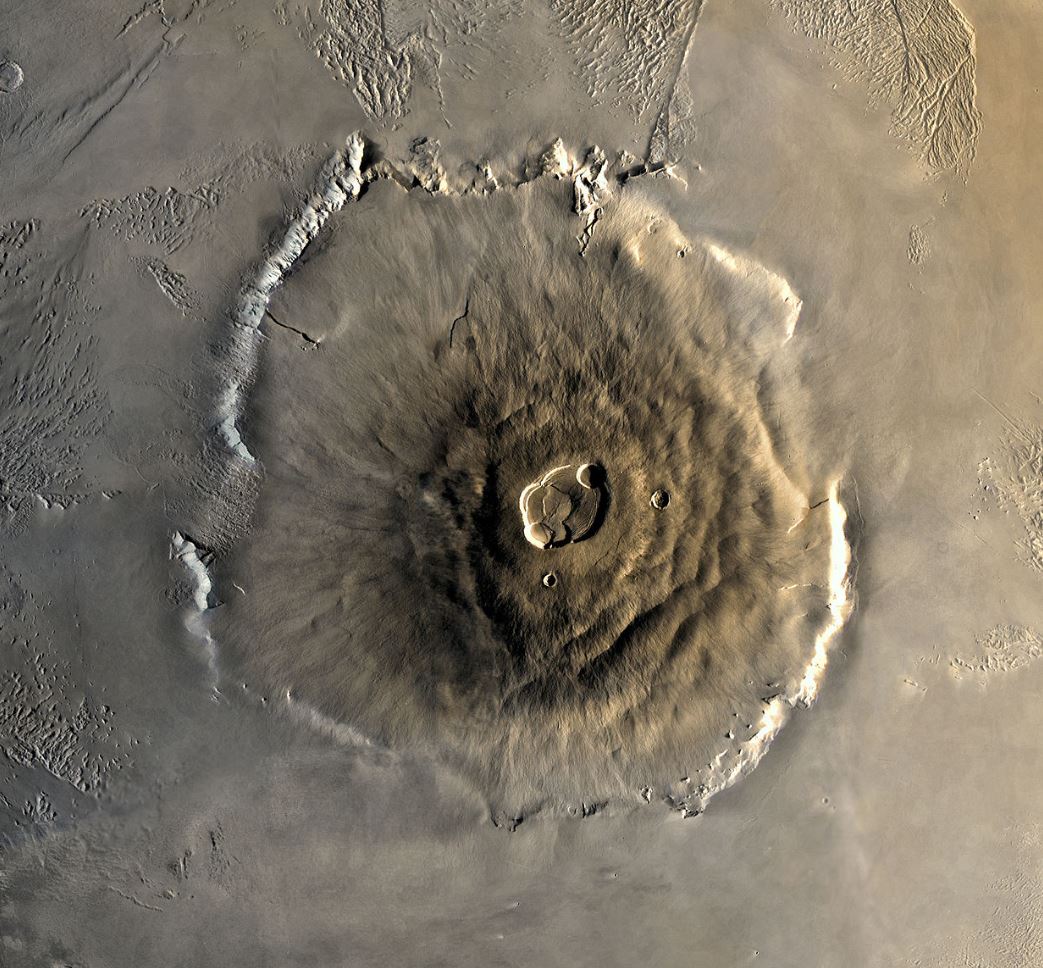
The largest volcanic edifice in the Solar System is Olympus Mons on Mars. This (possibly extinct) shield volcano covers an area the size of the state of Arizona. This may indicate the volcano erupted over a hotspot for millions of years, which means Mars had little, if any, plate tectonic activity.
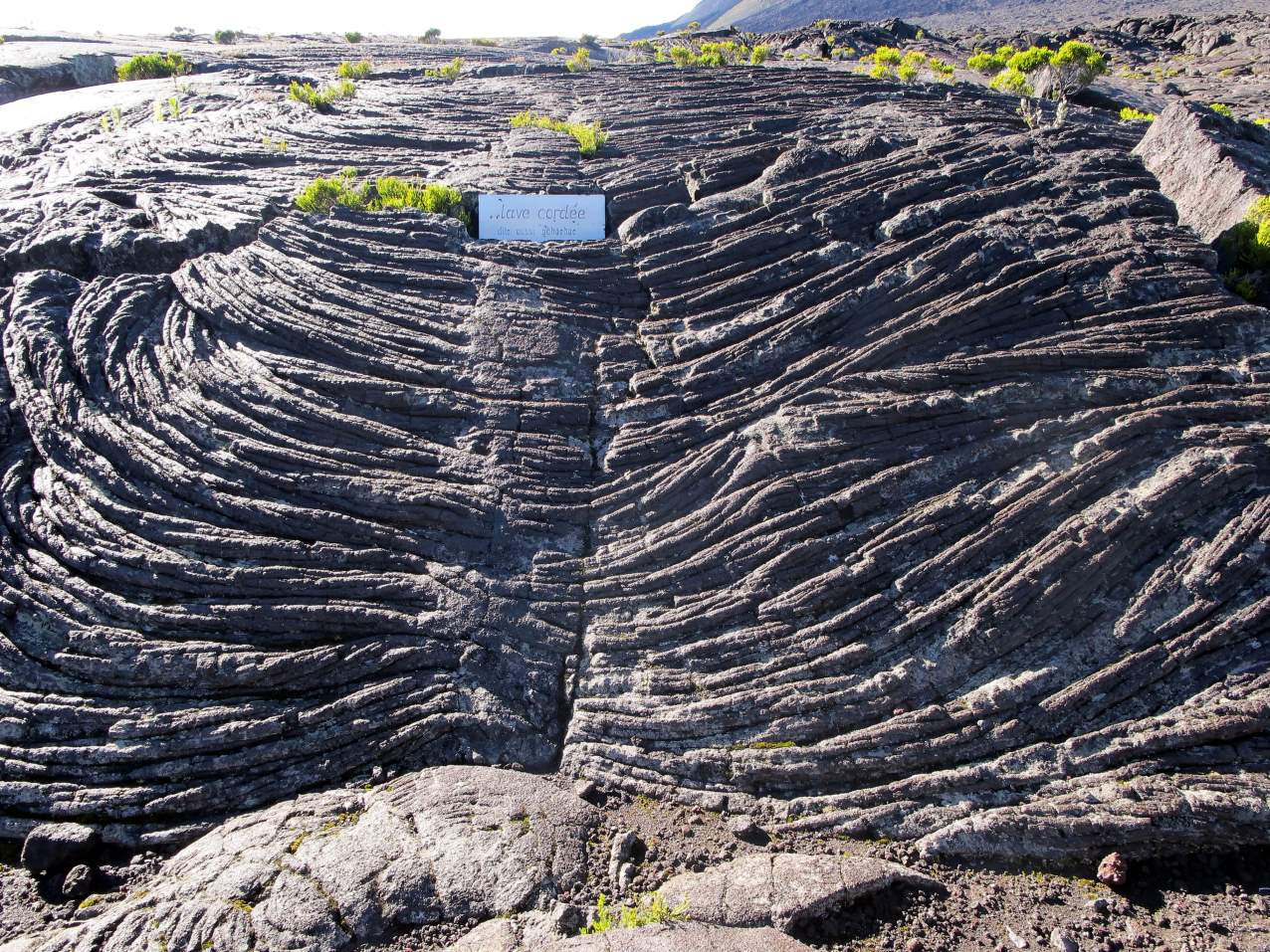
Basaltic lava forms special landforms based on magma temperature, composition, and content of dissolved gases and water vapor. The two main types of basaltic volcanic rock have Hawaiian names—pahoehoe and aa. Pahoehoe might come from low-viscosity lava that flows easily into ropey strands.
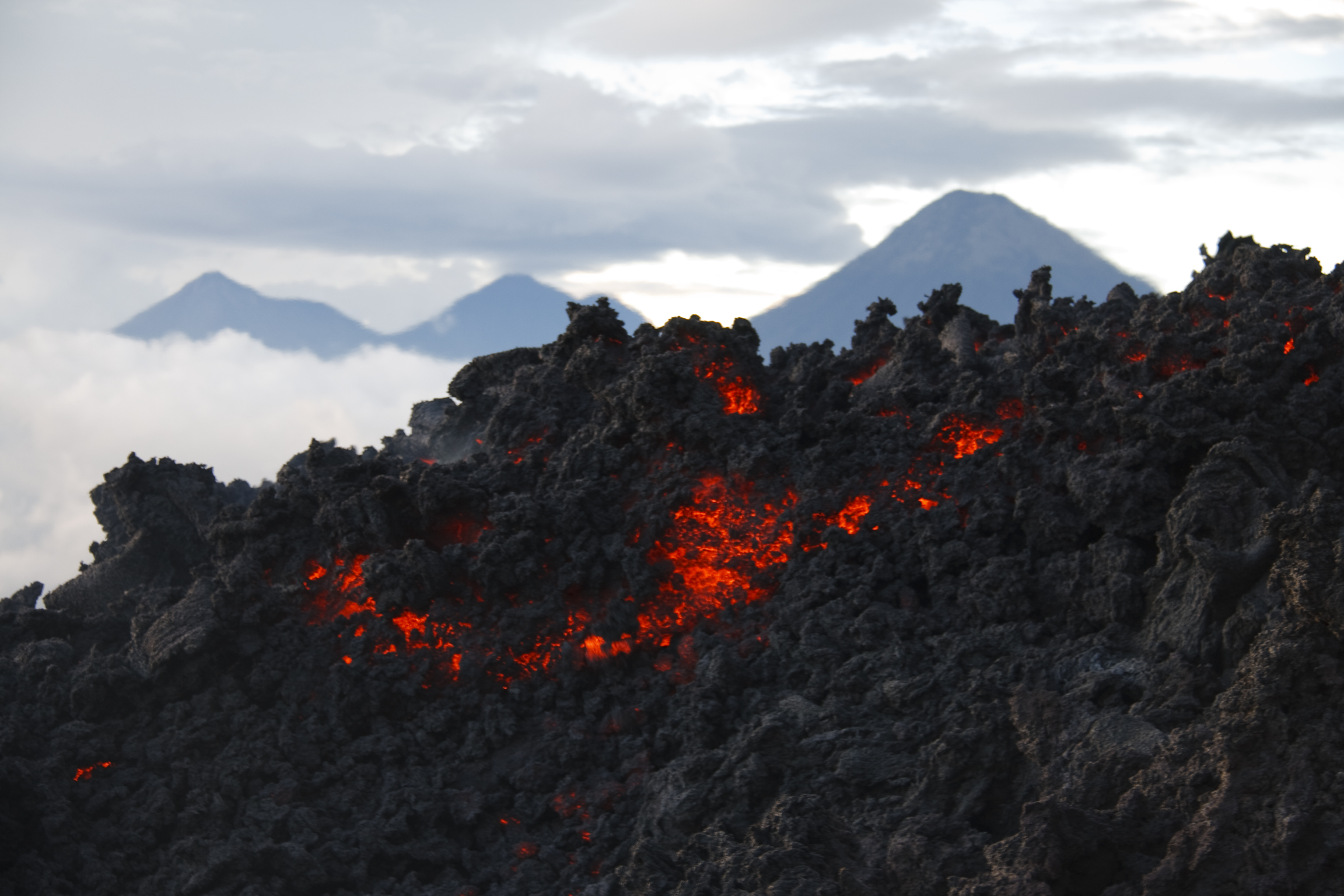
Aa (sometimes spelled a’a or ʻaʻā and pronounced “ah-ah”) is more viscous and has a crumbly blocky appearance. The exact details of what forms the two types of flows are still up for debate. Felsic lavas have lower temperatures and more silica, and thus are higher viscosity. These also form aa-style flows.
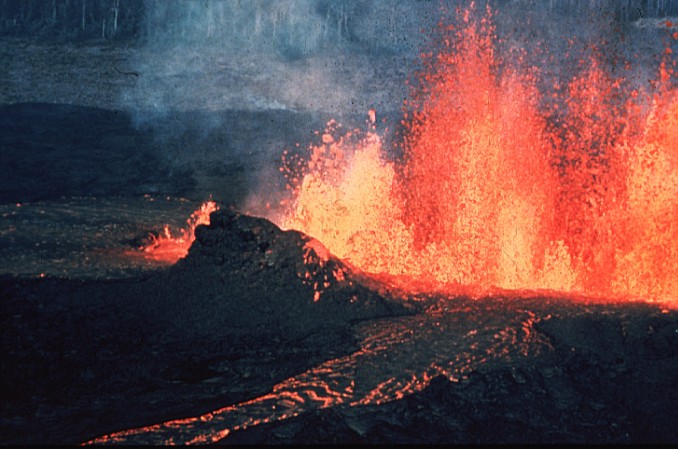
Low-viscosity, fast-flowing basaltic lava tends to harden on the outside into a tube and continue to flow internally. Once lava flow subsides, the empty outer shell may be left as a lava tube. Lava tubes, with or without collapsed roofs, make famous caves in Hawai’i, Northern California, the Columbia River Basalt Plateau of Washington and Oregon, El Malpais National Monument in New Mexico, and Craters of the Moon National Monument in Idaho.
Fissures are cracks that commonly originate from shield-style eruptions. Lava emerging from fissures is typically mafic and very fluid. The 2018 Kiluaea eruption included fissures associated with the lava flows. Some fissures are caused by the volcanic seismic activity rather than lava flows. Some fissures are influenced by plate tectonics, such as the common fissures located parallel to the divergent boundary in Iceland.
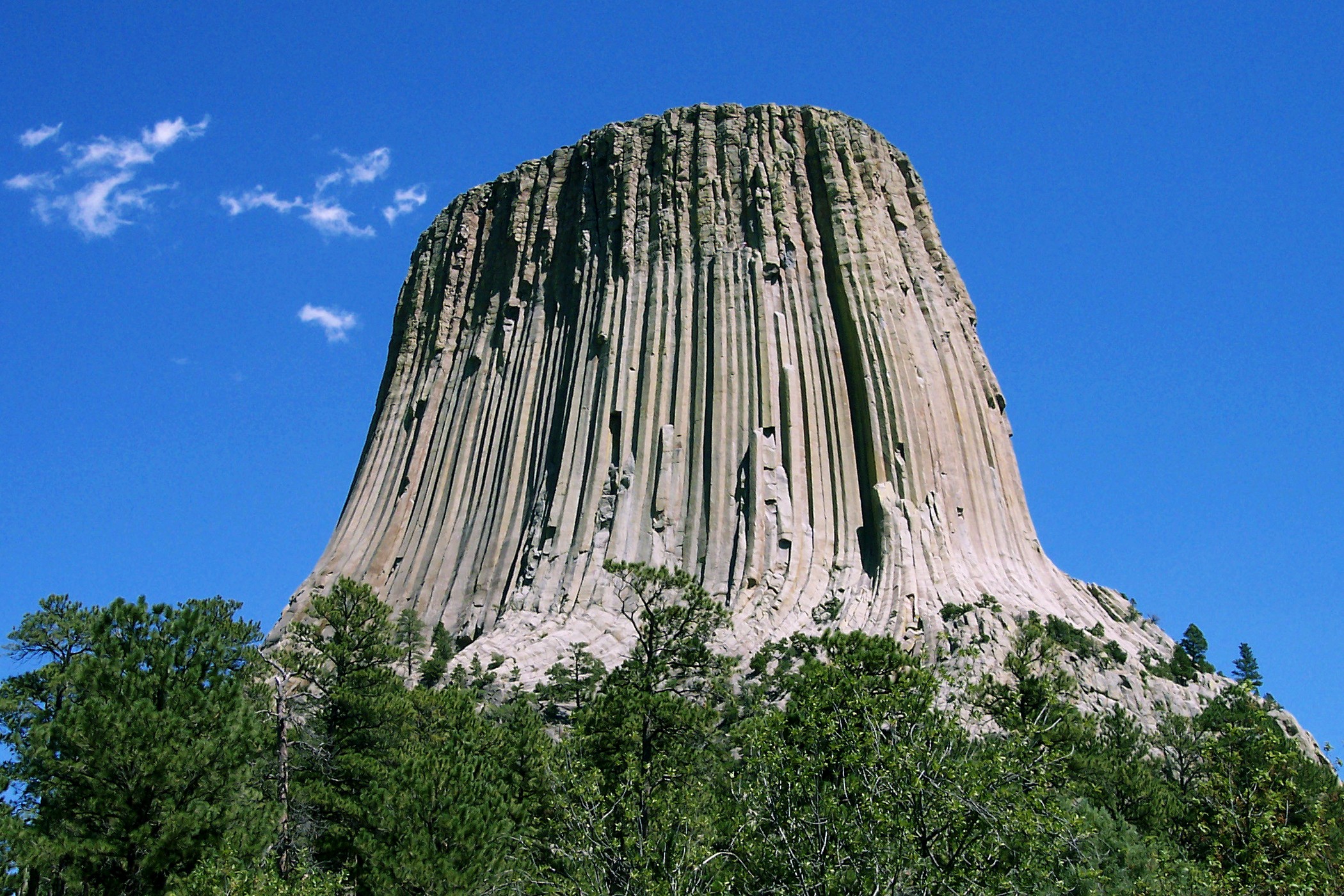
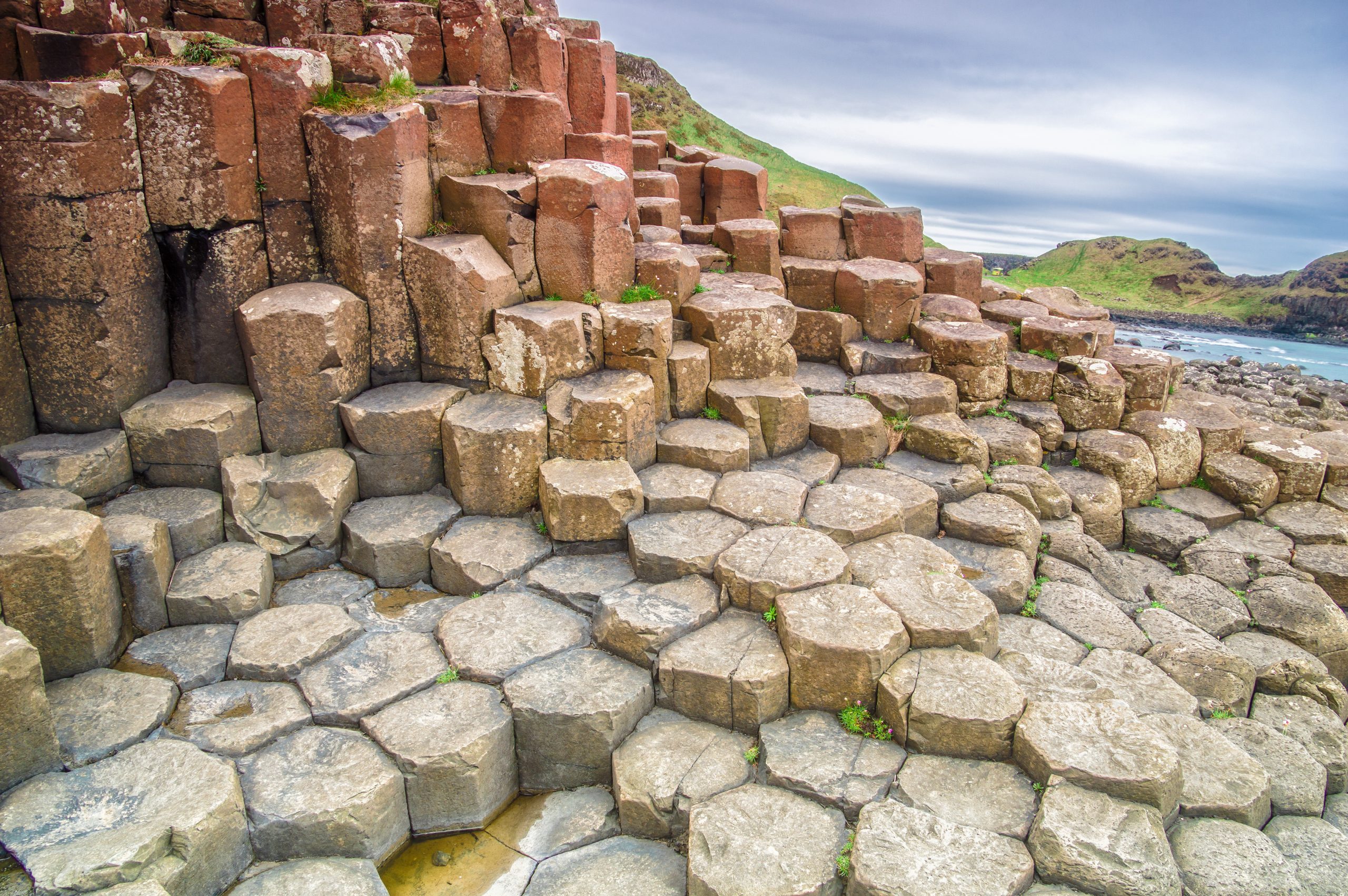
Cooling lava can contract into columns with semi-hexagonal cross sections called columnar jointing. This feature forms the famous Devils Tower in Wyoming, possibly an ancient volcanic vent from which the surrounding layers of lava and ash have been removed by erosion. Another well-known exposed example of columnar jointing is the Giant’s Causeway in Ireland.
Stratovolcano

A stratovolcano, also called a composite cone volcano, has steep flanks, a symmetrical cone shape, distinct crater, and rises prominently above the surrounding landscape. The term composite refers to the alternating layers of pyroclastic fragments like ash and bombs, and solidified lava flows of varying composition. Examples include Mount Rainier in Washington state and Mount Fuji in Japan.
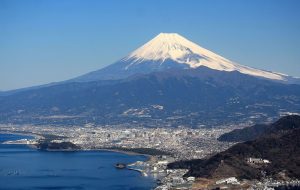
Stratovolcanoes usually have felsic to intermediate magma chambers, but can even produce mafic lavas. Stratovolcanoes have viscous lava flows and domes, punctuated by explosive eruptions. This produces volcanoes with steep flanks.
Lava Domes
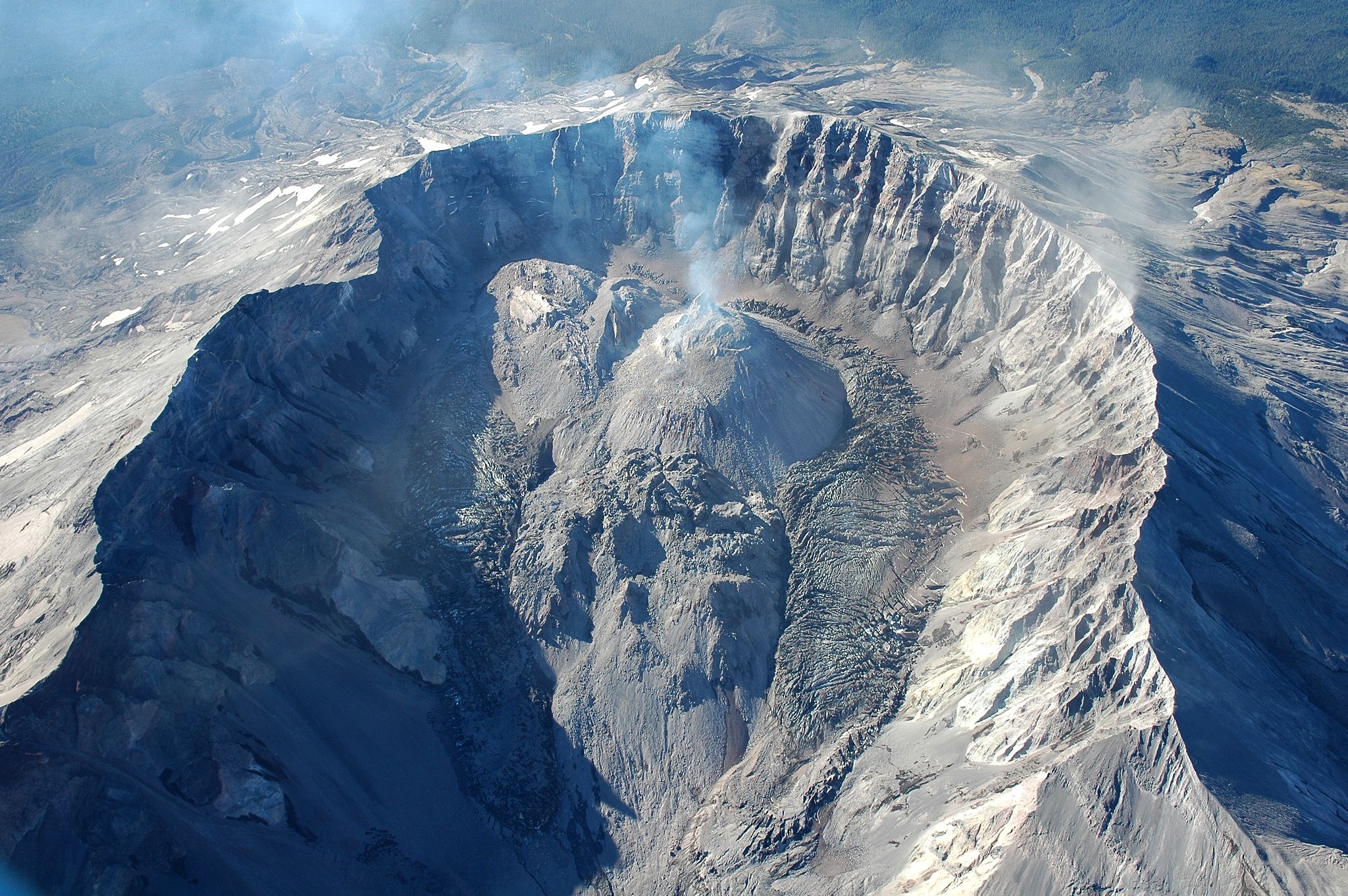
Lava domes are accumulations of silica-rich volcanic rock, such as rhyolite and obsidian. Too viscous to flow easily, the felsic lava tends to pile up near the vent in blocky masses. Lava domes often form in a vent within the collapsed crater of a stratovolcano, and grow by internal expansion. As the dome expands, the outer surface cools, hardens, and shatters, and spills loose fragments down the sides. Mount Saint Helens has a good example of a lava dome inside of a collapsed stratovolcano crater. Examples of stand-alone lava domes are Chaiten in Chile and Mammoth Mountain in California.
Caldera
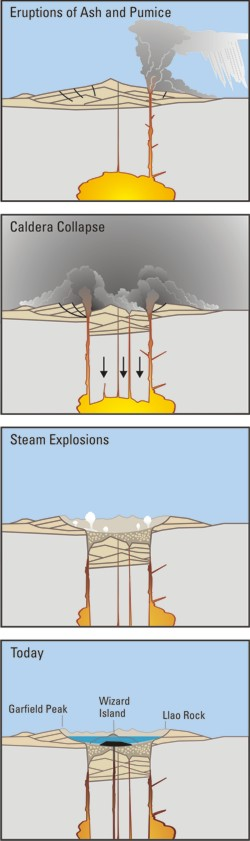
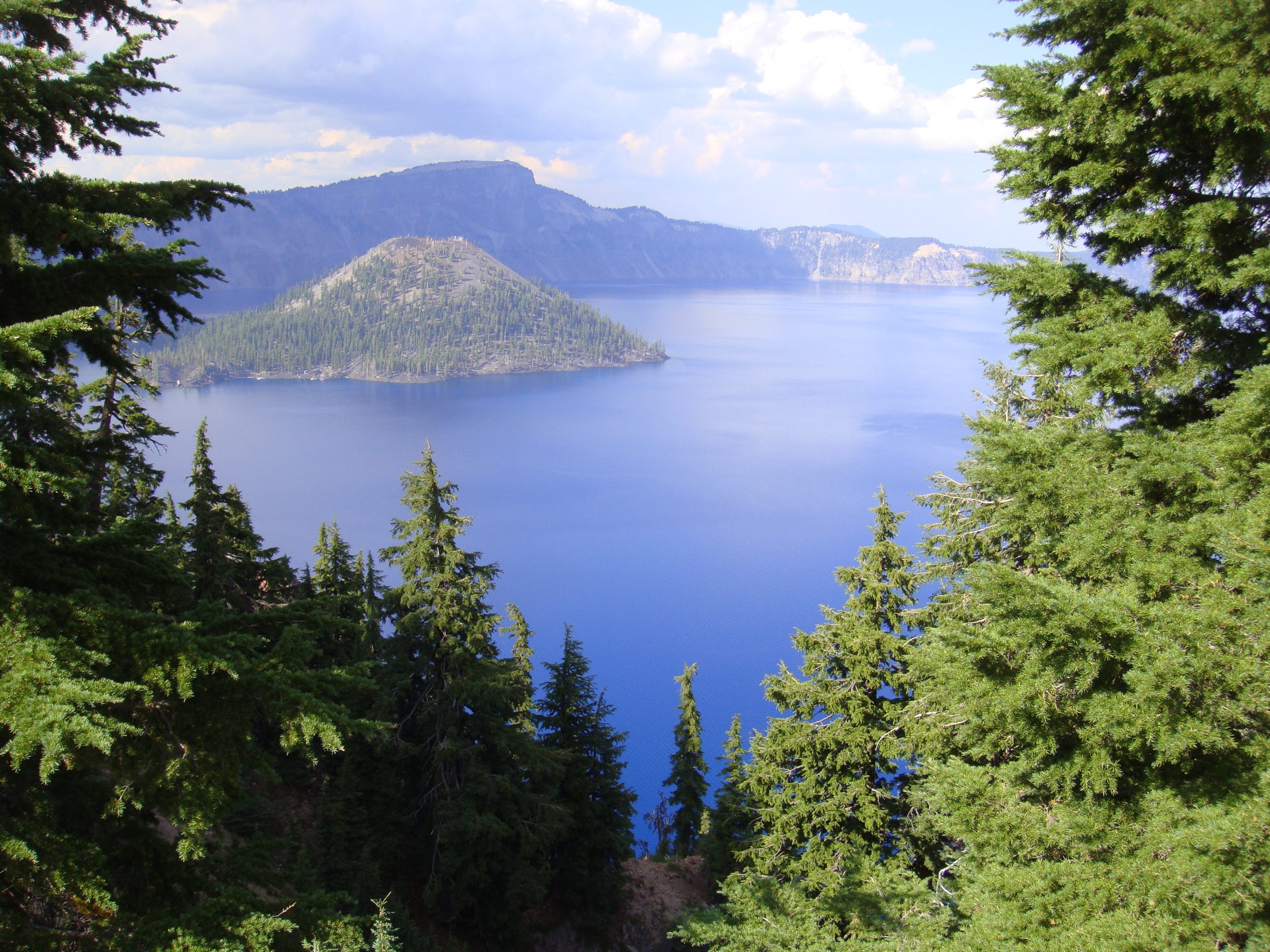
Calderas are steep-walled, basin-shaped depressions formed by the collapse of a volcanic edifice into an empty magma chamber. Calderas are generally very large, with diameters of up to 25 km (15.5 mi). The term caldera specifically refers to a volcanic vent; however, it is frequently used to describe a volcano type. Caldera volcanoes are typically formed by eruptions of high-viscosity felsic lava having high volatiles content.
Crater Lake, Yellowstone, and the Long Valley Caldera are good examples of this type of volcanism. The caldera at Crater Lake National Park in Oregon was created about 6,800 years ago when Mount Mazama, a composite volcano, erupted in a huge explosive blast. The volcano ejected large amounts of volcanic ash and rapidly drained the magma chamber, causing the top to collapse into a large depression that later filled with water. Wizard Island in the middle of the lake is a later resurgent lava dome that formed within the caldera basin.
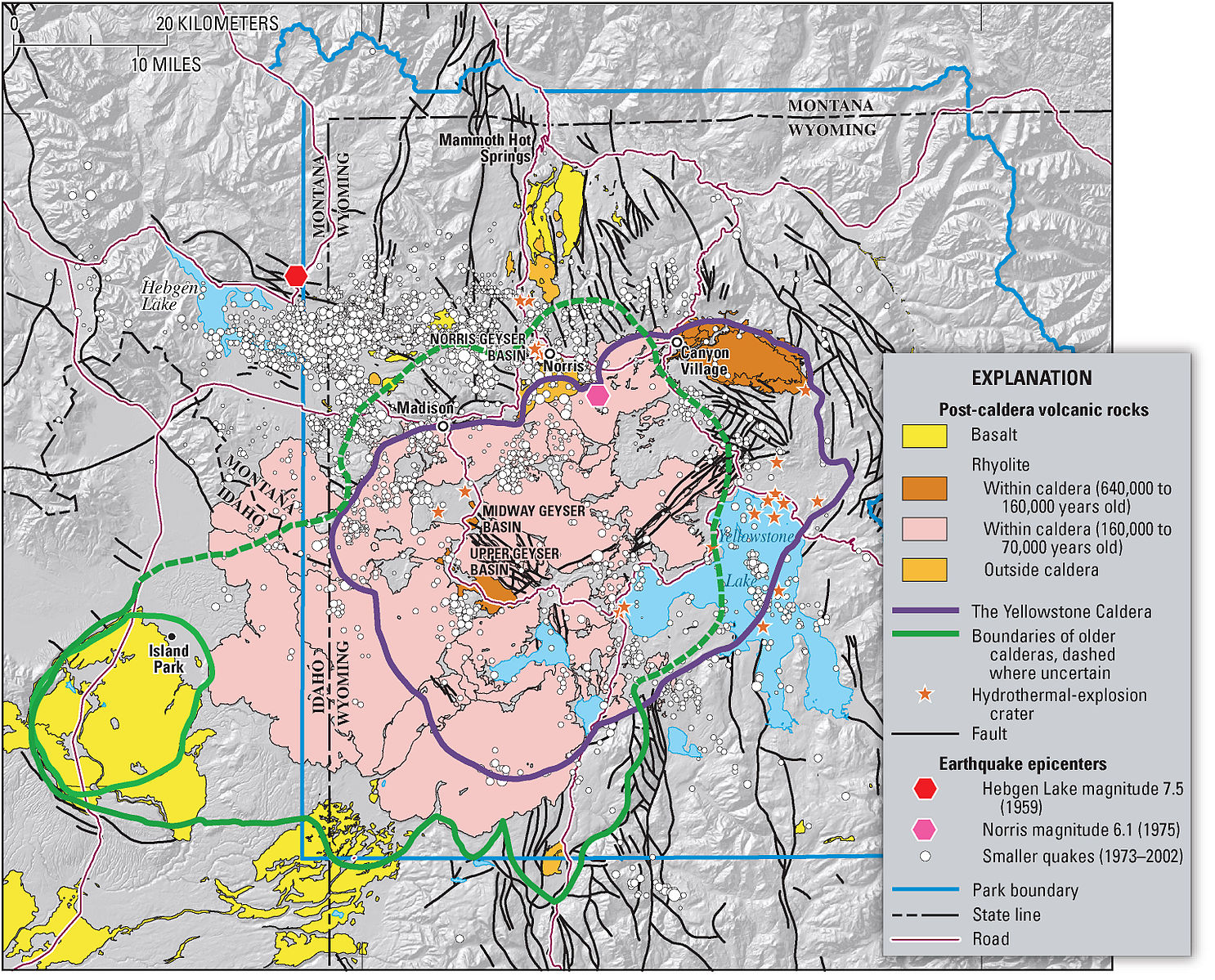
The Yellowstone volcanic system erupted three times in the recent geologic past—2.1, 1.3, and 0.64 million years ago—leaving behind three caldera basins. Each eruption created large rhyolite lava flows as well as pyroclastic flows that solidified into tuff formations. These extra-large eruptions rapidly emptied the magma chamber, causing the roof to collapse and form a caldera. The youngest of the three calderas contains most of Yellowstone National Park, as well as two resurgent lava domes. The calderas are difficult to see today due to the amount of time since their eruptions and subsequent erosion and glaciation.
Yellowstone volcanism started about 17-million years ago as a hotspot under the North American lithospheric plate near the Oregon/Nevada border. As the plate moved to the southwest over the stationary hotspot, it left behind a track of past volcanic activities. Idaho’s Snake River Plain was created from volcanism that produced a series of calderas and lava flows. The plate eventually arrived at its current location in northwestern Wyoming, where hotspot volcanism formed the Yellowstone calderas.
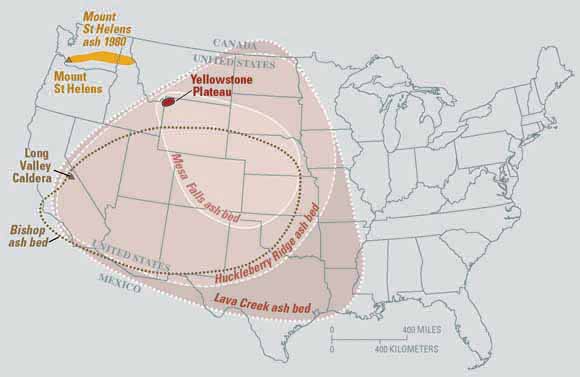
The Long Valley Caldera near Mammoth, California, is the result of a large volcanic eruption that occurred 760,000 years ago. The explosive eruption dumped enormous amounts of ash across the United States, in a manner similar to the Yellowstone eruptions. The Bishop Tuff deposit near Bishop, California, is made of ash from this eruption. The current caldera basin is 17 km by 32 km (10 mi by 20 mi), large enough to contain the town of Mammoth Lakes, major ski resort, airport, major highway, resurgent dome, and several hot springs.
Cinder Cone
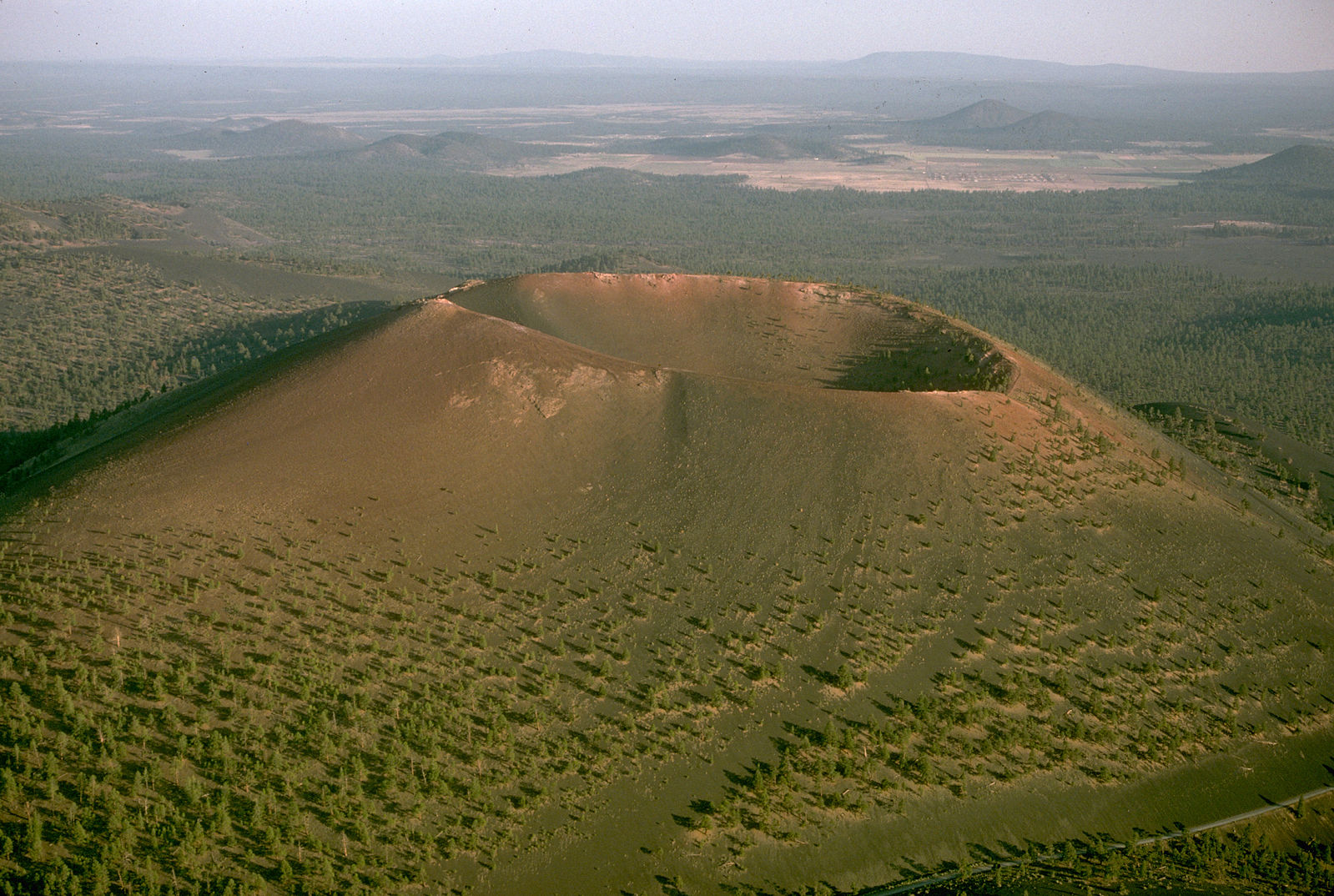
Cinder cones are small volcanoes with steep sides, and made of pyroclastic fragments that have been ejected from a pronounced central vent. The small fragments are called cinders and the largest are volcanic bombs. The eruptions are usually short-lived events, typically consisting of mafic lavas with a high content of volatiles. Hot lava is ejected into the air, cooling and solidifying into fragments that accumulate on the flank of the volcano. Cinder cones are found throughout western North America.
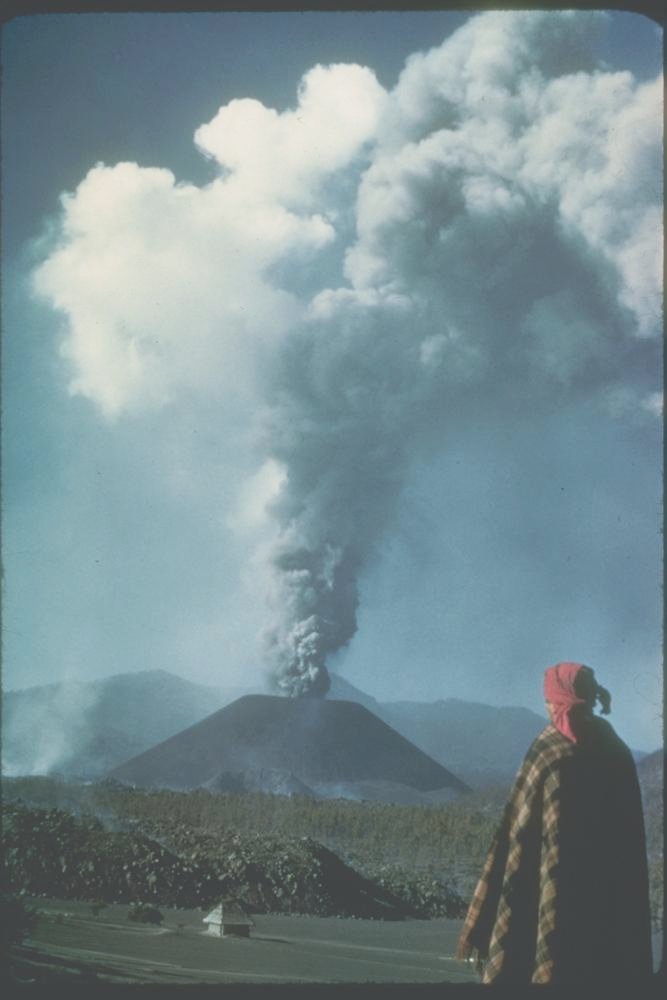
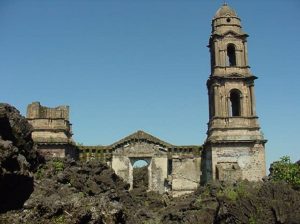
A recent and striking example of a cinder cone is the eruption near the village of Parícutin, Mexico that started in 1943. The cinder cone started explosively shooting cinders out of the vent in the middle of a farmer’s field. The volcanism quickly built up the cone to a height of over 90 m (300 ft) within a week, and 365 m (1,200 ft) within the first 8 months. After the initial explosive eruption of gases and cinders, basaltic lava poured out from the base of the cone. This is a common order of events for cinder cones: violent eruption, cone and crater formation, low-viscosity lava flow from the base. The cinder cone is not strong enough to support a column of lava rising to the top of the crater, so the lava breaks through and emerges near the bottom of the volcano. During nine years of eruption activity, the ashfall covered about 260 km2 (100 mi2) and destroyed the nearby town of San Juan.
Flood Basalts
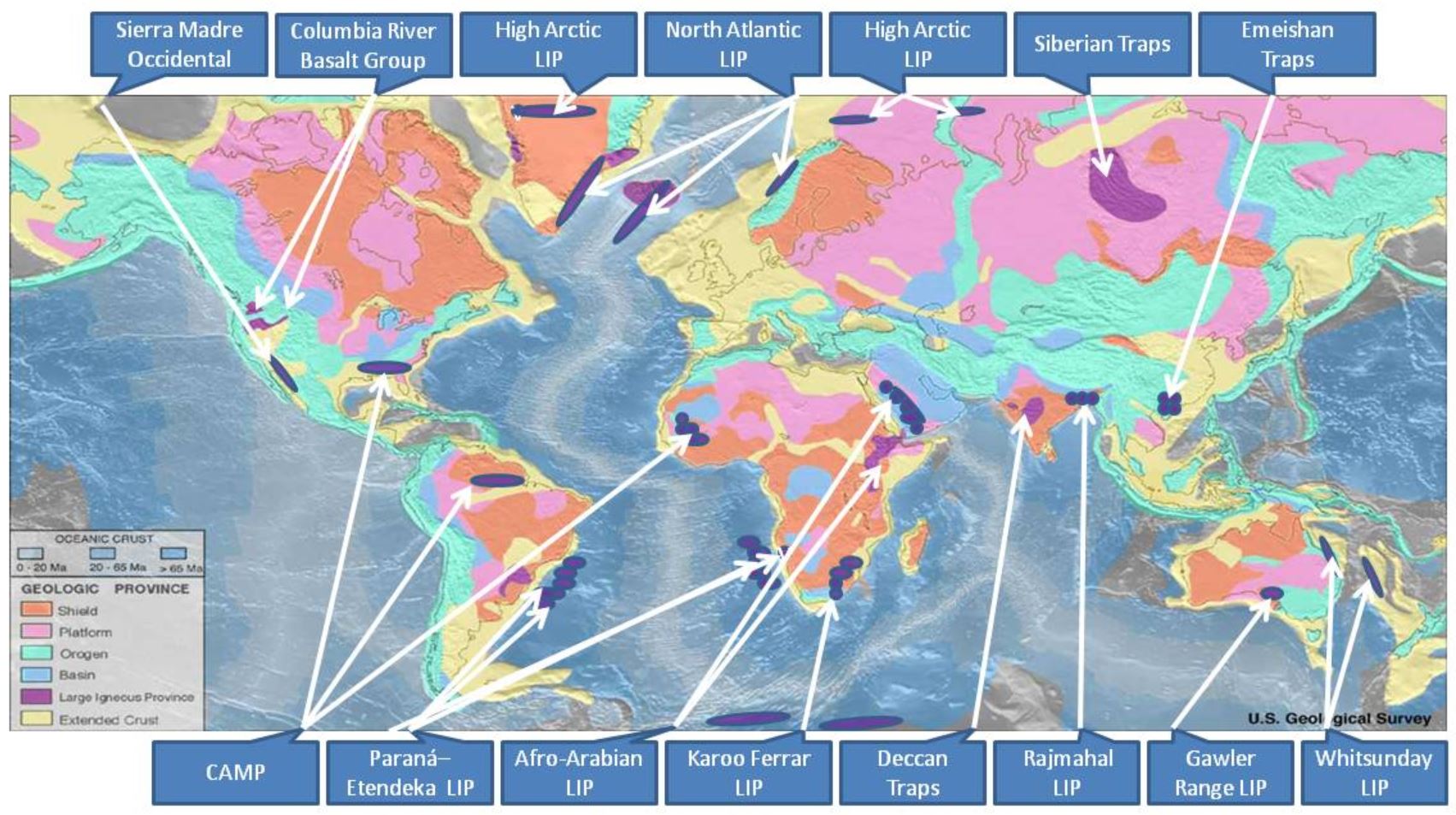
A rare volcanic eruption type, unobserved in modern times, is the flood basalt. Flood basalts are some of the largest and lowest viscosity types of eruptions known. They are not known from any eruption in human history, so the exact mechanisms of eruption are still mysterious. Some famous examples include the Columbia River Flood Basalts in Washington, Oregon, and Idaho, the Deccan Traps, which cover about 1/3 of the country of India, and the Siberian Traps, which may have been involved in the Earth’s largest mass extinction (see chapter 8).
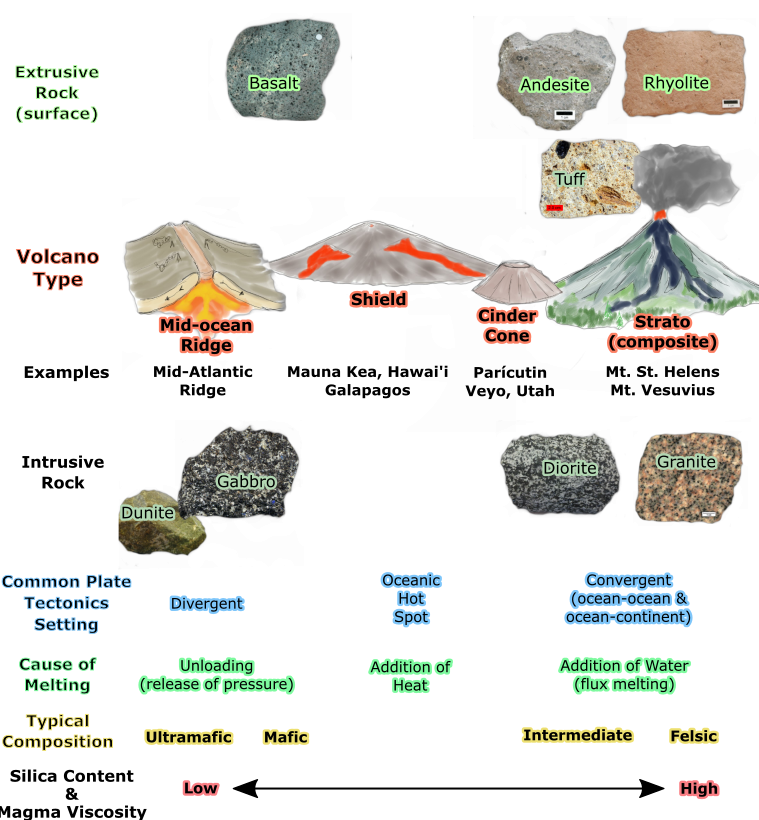
4.4.3 Volcanic Hazards and Monitoring
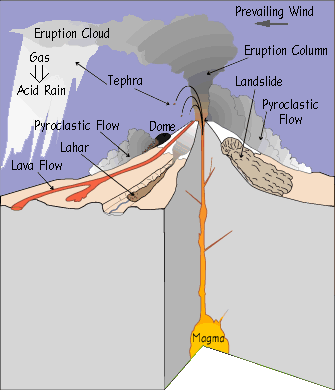
While the most obvious volcanic hazard is lava, the dangers posed by volcanoes go far beyond lava flows. For example, on May 18, 1980, Mount Saint Helens (Washington, United States) erupted with an explosion and landslide that removed the upper 400 m (1,300 ft) of the mountain. The initial explosion was immediately followed by a lateral blast, which produced a pyroclastic flow that covered nearly 600 km2 (230 mi2) of forest with hot ash and debris. The pyroclastic flow moved at speeds of 80-130 kph (50-80 mph), flattening trees and ejecting clouds of ash into the air. The USGS video provides an account of this explosive eruption that killed 57 people.
Video 4.6: Mout St. Helens.
If you are using an offline version of this text, access this YouTube video via the QR code.
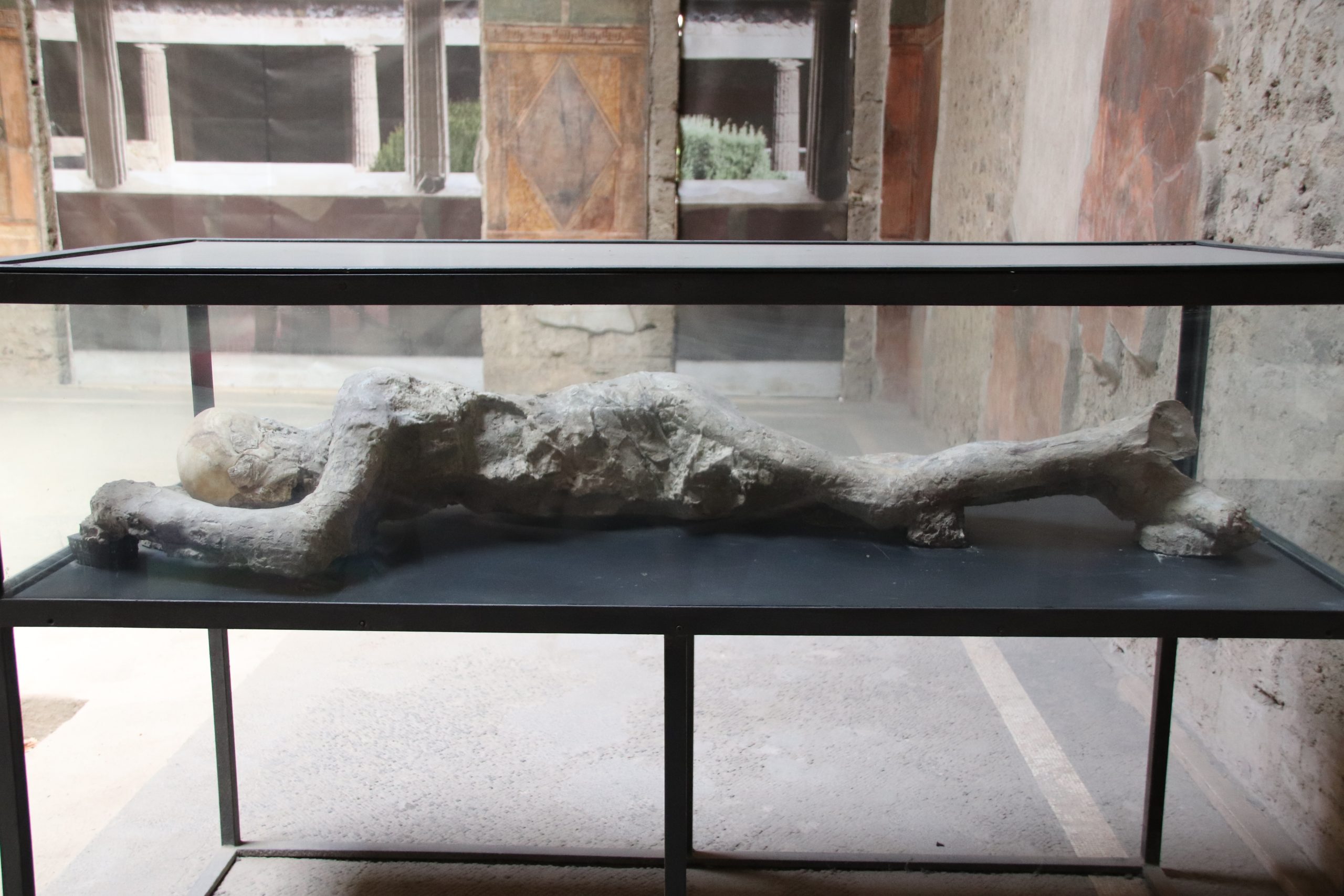
In 79 AD, Mount Vesuvius, located near Naples, Italy, violently erupted sending a pyroclastic flow over the Roman countryside, including the cities of Herculaneum and Pompeii. The buried towns were discovered in an archeological expedition in the 18th century. Pompeii famously contains the remains (casts) of people suffocated by ash and covered by 10 feet (3 m) of ash, pumice lapilli, and collapsed roofs.
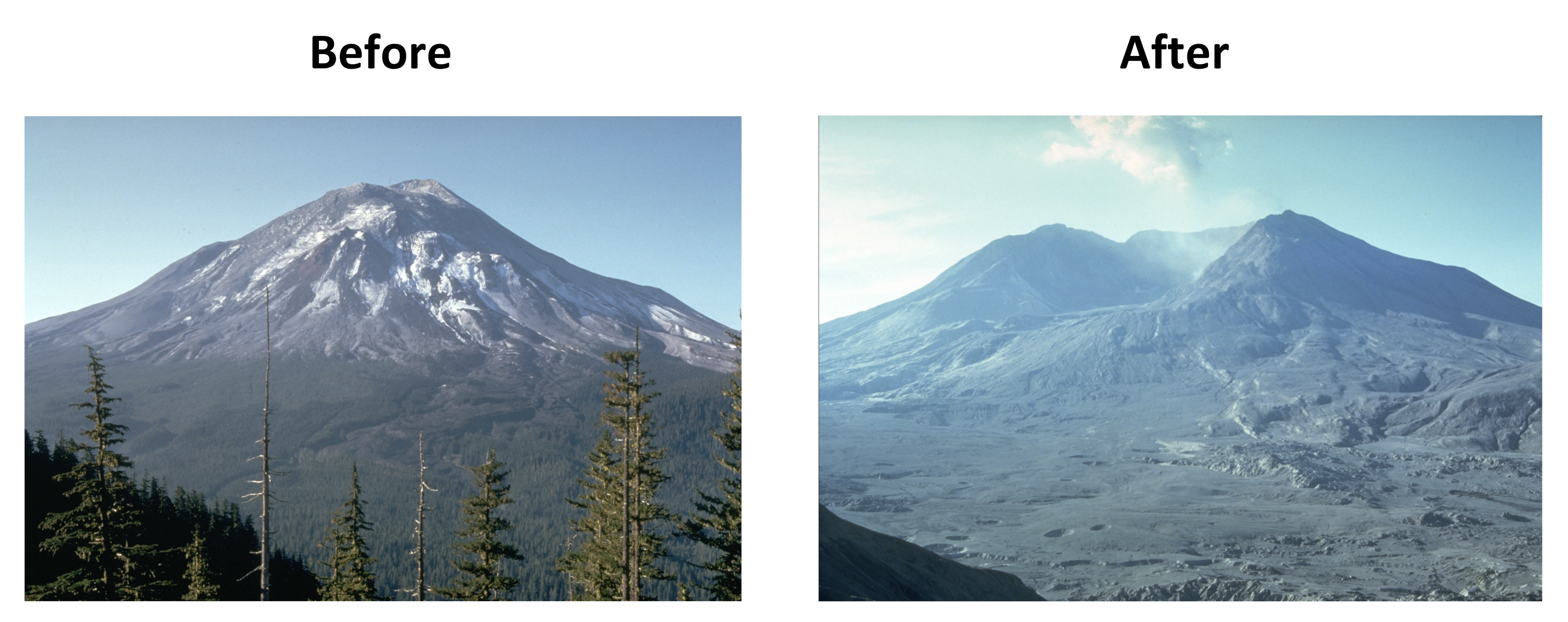
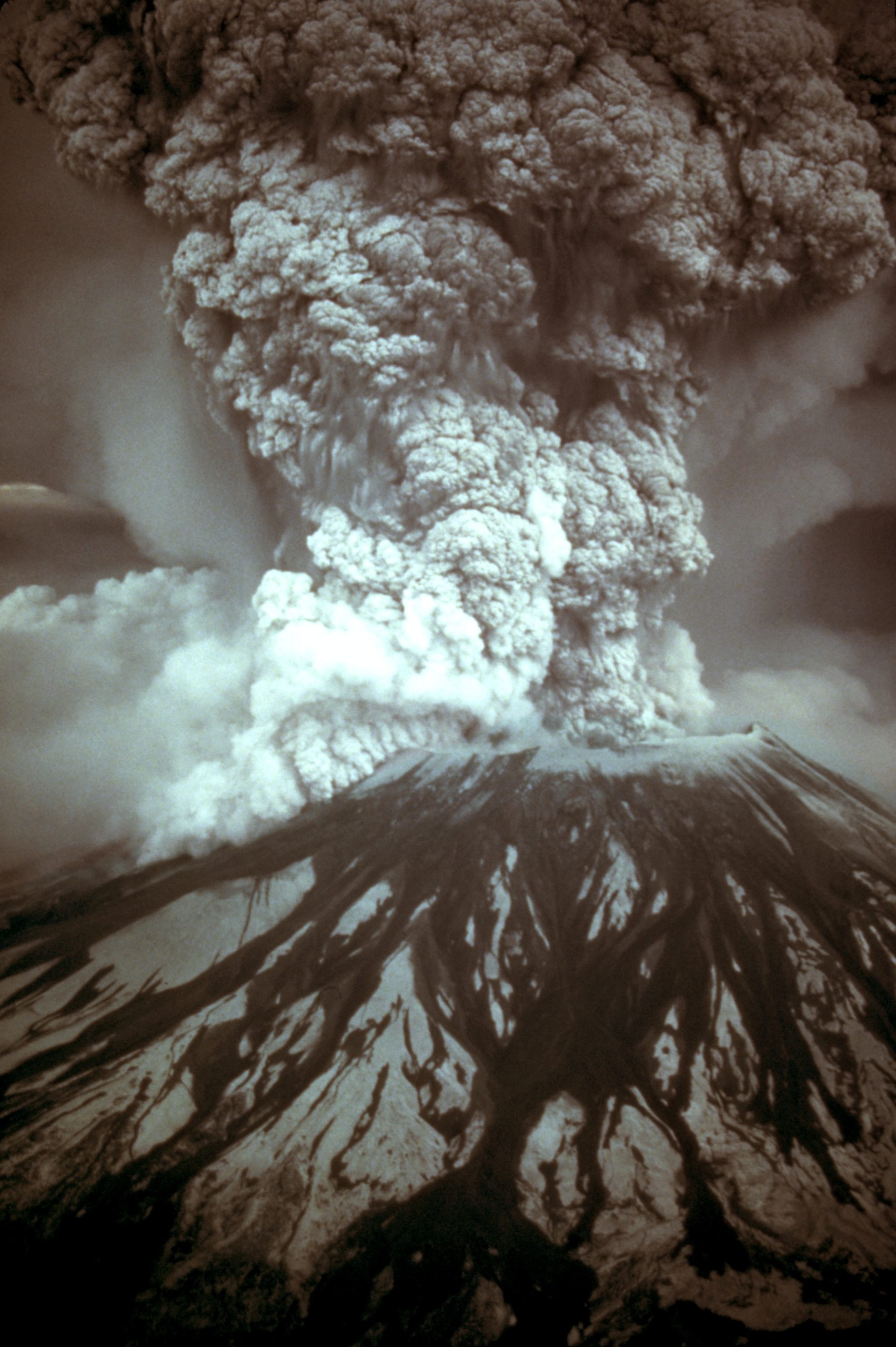
Pyroclastic Flows
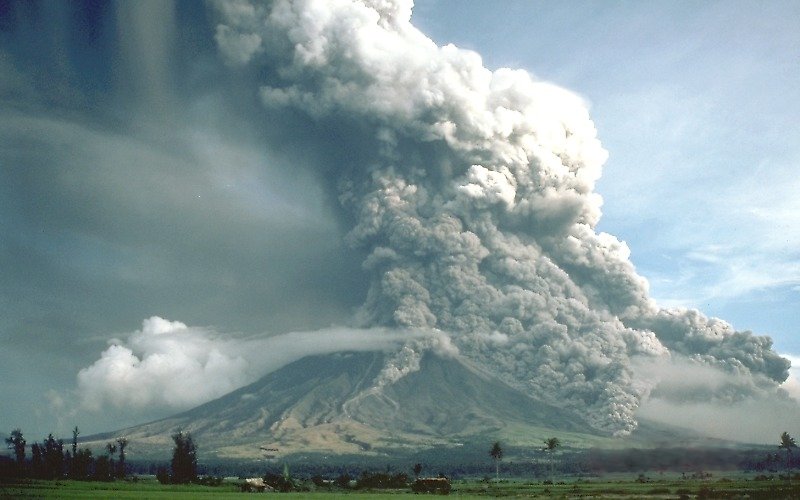
The most dangerous volcanic hazard are pyroclastic flows (video). These flows are a mix of lava blocks, pumice, ash, and hot gases between 200°C-700°C (400°F-1,300°F). The turbulent cloud of ash and gas races down the steep flanks at high speeds up to 193 kph (120 mph) into the valleys around composite volcanoes. Most explosive, silica-rich, high viscosity magma volcanoes such as composite cones usually have pyroclastic flows. The rock tuff and welded tuff is often formed from these pyroclastic flows.
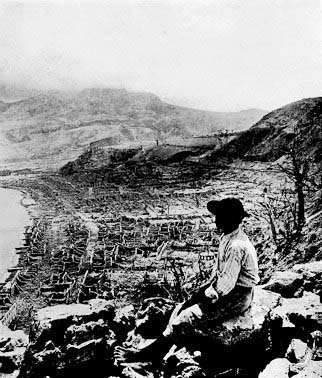
There are numerous examples of deadly pyroclastic flows. In 2014, the Mount Ontake pyroclastic flow in Japan killed 47 people. The flow was caused by magma heating groundwater into steam, which then rapidly ejected with ash and volcanic bombs. Some were killed by inhalation of toxic gases and hot ash, while others were struck by volcanic bombs. Two short videos below document eye-witness video of pyroclastic flows. In the early 1990s, Mount Unzen erupted several times with pyroclastic flows. The pyroclastic flow shown in this famous short video killed 41 people. In 1902, on the Caribbean Island Martinique, Mount Pelee erupted with a violent pyroclastic flow that destroyed the entire town of St. Pierre and killing 28,000 people in moments.
Video 4.7: Dome collapse and pyroclastic flow at Unzen Volcano.
If you are using an offline version of this text, access this YouTube video via the QR code.
Landslides and Landslide–Generated Tsunamis
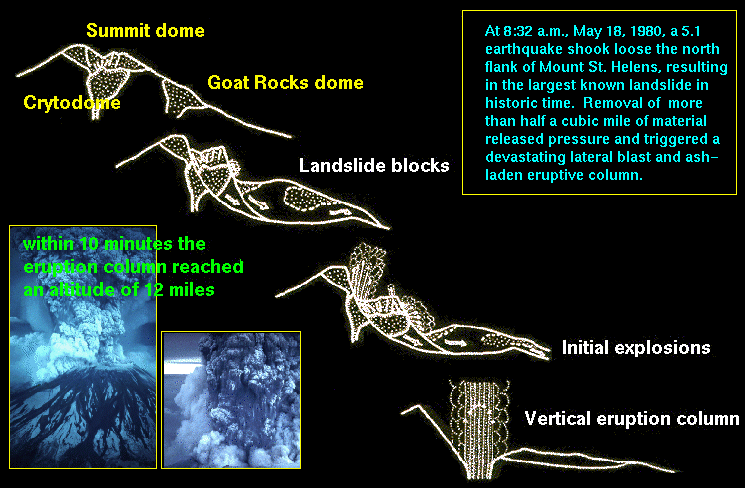
The steep and unstable flanks of a volcano can lead to slope failure and dangerous landslides. These landslides can be triggered by magma movement, explosive eruptions, large earthquakes, and/or heavy rainfall. During the 1980 Mount St. Helens eruption, the entire north flank of the volcano collapsed and released a huge landslide that moved at speeds of 160-290 kph (100-180 mph).
If enough landslide material reaches the ocean, it may cause a tsunami. In 1792, a landslide caused by the Mount Unzen eruption reached the Ariaka Sea, generating a tsunami that killed 15,000 people (see USGS page). When Mount Krakatau in Indonesia erupted in 1883, it generated ocean waves that towered 40 m (131 ft) above sea level. The tsunami killed 36,000 people and destroyed 165 villages.
Tephra
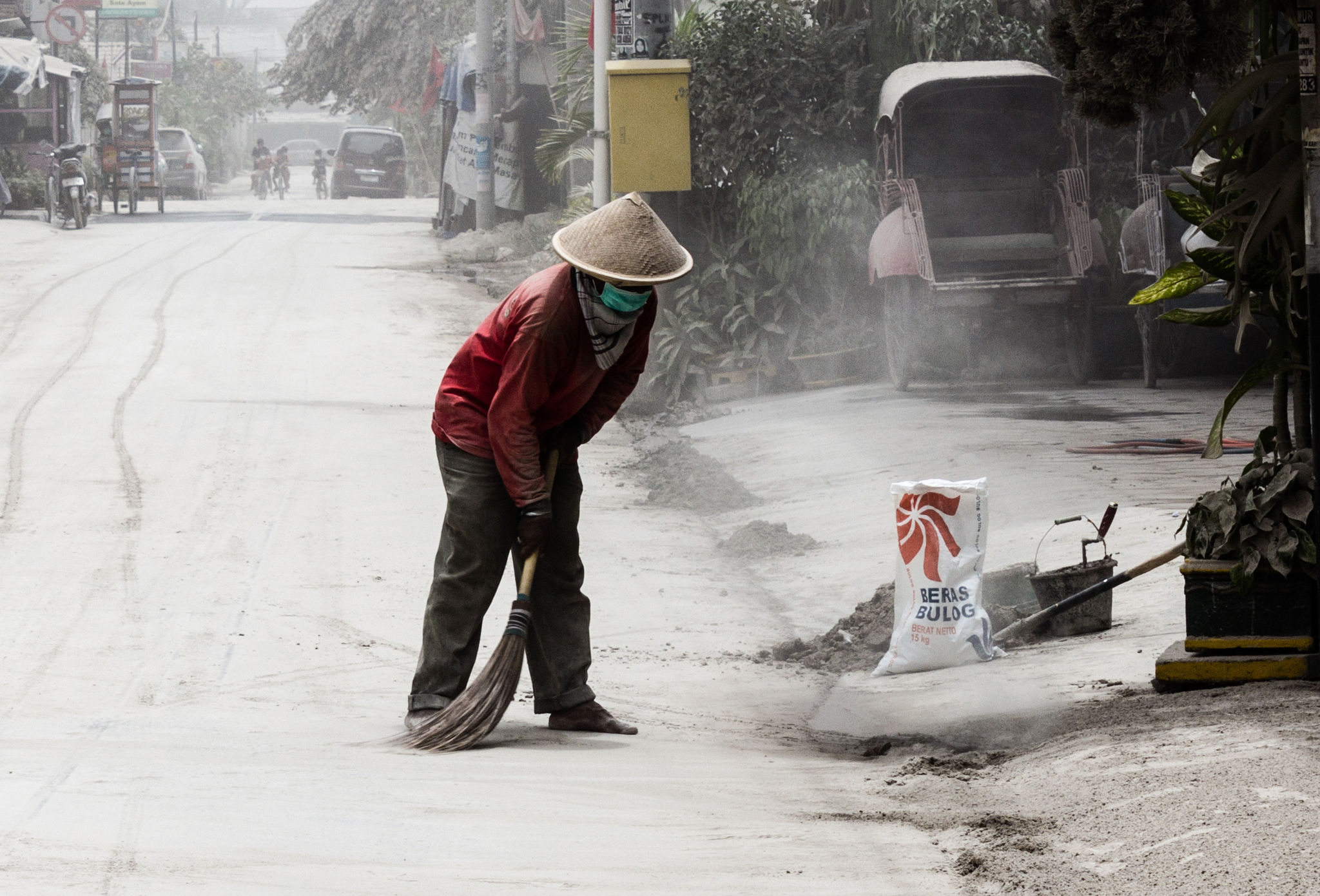
Volcanoes, especially composite volcanoes, eject large amounts of tephra (ejected rock materials), most notably ash (tephra fragments less than 0.08 inches [2 mm]). Larger tephra is heavier and falls closer to the vent. Larger blocks and bombs pose hazards to those close to the eruption such as at the 2014 Mount Ontake disaster in Japan discussed earlier.
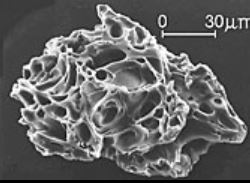
Hot ash poses an immediate danger to people, animals, plants, machines, roads, and buildings located close to the eruption. Ash is fine grained (< 2mm) and can travel airborne long distances away from the eruption site. Heavy accumulations of ash can cause buildings to collapse. In people, it may cause respiratory issues like silicosis. Ash is destructive to aircraft and automobile engines, which can disrupt transportation and shipping services. In 2010, the Eyjafjallajökull volcano in Iceland emitted a large ash cloud into the upper atmosphere, causing the largest air-travel disruption in northern Europe since World War II. No one was injured, but the service disruption was estimated to have cost the world economy billions of dollars.
Volcanic Gases
As magma rises to the surface the confining pressure decreases, and allows dissolved gases to escape into the atmosphere. Even volcanoes that are not actively erupting may emit hazardous gases, such as carbon dioxide (CO2), sulfur dioxide (SO2), hydrogen sulfide (H2S), and hydrogen halides (HF, HCl, or HBr).
Carbon dioxide tends to sink and accumulate in depressions and basins. In volcanic areas known to emit carbon dioxide, low-lying areas may trap hazardous concentrations of this colorless and odorless gas. The Mammoth Mountain Ski Resort in California, is located within the Long Valley Caldera, is one such area of carbon dioxide-producing volcanism. In 2006, three ski patrol members died of suffocation caused by carbon dioxide after falling into a snow depression near a fumarole (info).
In rare cases, volcanism may create a sudden emission of gases without warning. Limnic eruptions (limne is Greek for lake), occur in crater lakes associated with active volcanism. The water in these lakes is supercharged with high concentrations of dissolved gases. If the water is physically jolted by a landslide or earthquake, it may trigger an immediate and massive release of gases out of solution. An analogous example would be what happens to vigorously shaken bottle of carbonated soda when the cap is opened. An infamous limnic eruption occurred in 1986 at Lake Nyos, Cameroon. Almost 2,000 people were killed by a massive release of carbon dioxide.
Lahars
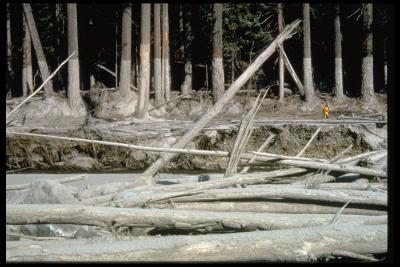
Lahar is an Indonesian word and is used to describe a volcanic mudflow that forms from rapidly melting snow or glaciers. Lahars are slurries resembling wet concrete, and consist of water, ash, rock fragments, and other debris. These mudflows flow down the flanks of volcanoes or mountains covered with freshly-erupted ash and on steep slopes can reach speeds of up to 80 kph (50 mph).
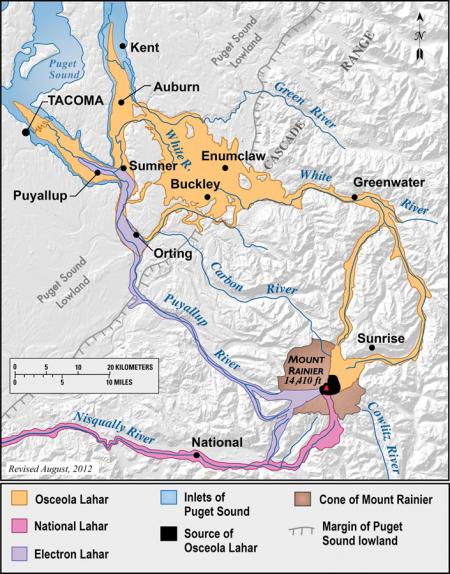
Several major cities, including Tacoma, are located on prehistoric lahar flows that extend for many kilometers across the flood plains surrounding Mount Rainier in Washington (see map). A map of Mount Baker in Oregon shows a similar potential hazard for lahar flows (see map). A tragic scenario played out recently, in 1985, when a lahar from the Nevado del Ruiz volcano in Colombia buried the town of Armero and killed an estimated 23,000 people.
Monitoring
Geologists use various instruments to detect changes or indications that an eruption is imminent. The three videos show different types of volcanic monitoring used to predict eruptions 1) earthquake activity; 2) increases in gas emission; and 3) changes in land surface orientation and elevation.
One video shows how monitoring earthquake frequency, especially special vibrational earthquakes called harmonic tremors, can detect magma movement and possible eruption. Another video shows how gas monitoring may be used to predict an eruption. A rapid increase of gas emission may indicate magma that is actively rising to surface and releasing dissolved gases out of solution, and that an eruption is imminent. The last video shows how a GPS unit and tiltmeter can detect land surface changes, indicating the magma is moving underneath it.
Video 4.8: Earthquake signals.
If you are using an offline version of this text, access this YouTube video via the QR code.
Video 4.9: Measuring gas emissions.
If you are using an offline version of this text, access this YouTube video via the QR code.
Video 4.10: Using tiltmeters and GPS to monitor a volcano.
If you are using an offline version of this text, access this YouTube video via the QR code.
Take this quiz to check your comprehension of this section.
If you are using an offline version of this text, access the quiz for section 4.4 via the QR code.
Summary
Igneous rock is divided into two major groups: intrusive rock that solidifies from underground magma, and extrusive rock formed from lava that erupts and cools on the surface. Magma is generated from mantle material at several plate tectonics situations by three types of melting: decompression melting, flux melting, or heat-induced melting. Magma composition is determined by differences in the melting temperatures of the mineral components (Bowen’s Reaction Series). The processes affecting magma composition include partial melting, magmatic differentiation, assimilation, and collision. Volcanoes come in a wide variety of shapes and sizes, and are classified by a multiple factors, including magma composition, and plate tectonic activity. Because volcanism presents serious hazards to human civilization, geologists carefully monitor volcanic activity to mitigate or avoid the dangers it presents.
Take this quiz to check your comprehension of this chapter.
If you are using an offline version of this text, access the quiz for chapter 4 via the QR code.
URLs Listed Within This Chapter
USGS page of Mauna Loa: https://www.usgs.gov/volcanoes/mauna-loa
USGS page on Kilauea: https://www.usgs.gov/volcanoes/kilauea
Pyroclastic flows video: https://volcanoes.usgs.gov/vsc/movies/movie_101/PF_Animation.mp4
USGS page on volcano landslides that trigger waves and tsunamis: https://volcanoes.usgs.gov/Imgs/Jpg/Unzen/MayuyamaSlide_caption.html
Ski patrol’s fatal fall into a volcanic fumarole: https://pubmed.ncbi.nlm.nih.gov/19364170/
Text References
- Arndt, N.T., 1994, Chapter 1 Archean Komatiites, in K.C. Condie, editor, Developments in Precambrian Geology: Elsevier, p. 11–44.
- Bateman, P.C., and Chappell, B.W., 1979, Crystallization, fractionation, and solidification of the Tuolumne Intrusive Series, Yosemite National Park, California: Geological Society of America Bulletin, v. 90, no. 5, p. 465–482., https://doi.org/10.1130/0016-7606(1979)90<465:CFASOT>2.0.CO;2
- Boehler, R., 1996, Melting temperatures of the Earth’s mantle and core: Earth’s thermal structure: Annual Review of Earth and Planetary Sciences, v. 24, no. 1, p. 15–40., doi: 10.1146/annurev.earth.24.1.15.
- Bowen, N.L., 1922, The Reaction Principle in Petrogenesis: J. Geol., v. 30, no. 3, p. 177–198.
- Bowen, N.L., 1928, The evolution of the igneous rocks: Dover Publications, 334 p.
- Carr, M.H., 1975, Geologic map of the Tharsis Quadrangle of Mars: IMAP.
- Earle, S., 2015, Physical geology OER textbook: BC Campus OpenEd.
- EarthScope, 2014, Mount Ontake Volcanic Eruption: Online, http://www.earthscope.org/science/geo-events/mount-ontake-volcanic-eruption, accessed July 2016.
- Frankel, C., 2005, Worlds on Fire: Volcanoes on the Earth, the Moon, Mars, Venus and Io: Cambridge University Press, 396 p.
- Glazner, A.F., Bartley, J.M., Coleman, D.S., Gray, W., and Taylor, R.Z., 2004, Are plutons assembled over millions of years by amalgamation from small magma chambers? GSA Today, v. 14, no. 4, p. 4., DOI: 10.1130/1052-5173(2004)014<0004:APAOMO>2.0.CO;2
- Luongo, G., Perrotta, A., Scarpati, C., De Carolis, E., Patricelli, G., and Ciarallo, A., 2003, Impact of the AD 79 explosive eruption on Pompeii, II. Causes of death of the inhabitants inferred by stratigraphic analysis and areal distribution of the human casualties: J. Volcanol. Geotherm. Res., v. 126, no. 3–4, p. 169–200.
- Mueller, S., and Phillips, R.J., 1991, On the initiation of subduction: J. Geophys. Res. [Solid Earth], v. 96, no. B1, p. 651–665.
- Peacock, M.A., 1931, Classification of Igneous Rock Series: The Journal of Geology, v. 39, no. 1, p. 54–67.
- Perkins, S., 2011, 2010’s Volcano-Induced Air Travel Shutdown Was Justified: Online, http://www.sciencemag.org/news/2011/04/2010s-volcano-induced-air-travel-shutdown-was-justified, accessed July 2016.
- Peterson, D.W., and Tilling, R.I., 1980, Transition of basaltic lava from pahoehoe to aa, Kilauea Volcano, Hawaii: Field observations and key factors – ScienceDirect: J. Volcanol. Geotherm. Res., v. 7, no. 3–4, p. 271–293.
- Petrini and Podladchikov, 2000, Lithospheric pressure–depth relationship in compressive regions of thickened crust: Journal of Metamorphic Geology, v. 18, no. 1, p. 67–77., doi: 10.1046/j.1525-1314.2000.00240.x.
- Reid, J.B., Evans, O.C., and Fates, D.G., 1983, Magma mixing in granitic rocks of the central Sierra Nevada, California: Earth and Planetary Science Letters, v. 66, p. 243–261., doi: 10.1016/0012-821X(83)90139-5.
- Rhodes, J.M., and Lockwood, J.P., 1995, Mauna Loa Revealed: Structure, Composition, History, and Hazards: Washington DC American Geophysical Union Geophysical Monograph Series, v. 92.
- Scandone, R., Giacomelli, L., and Gasparini, P., 1993, Mount Vesuvius: 2000 years of volcanological observations: Journal of Volcanology and Geothermal Research, v. 58, p. 5–25.
- Stovall, W.K., Wilkins, A.M., Mandeville, C.W., and Driedger, C.L., 2016, Fact Sheet.
- Thorarinsson, S., 1969, The Lakagigar eruption of 1783: Bull. Volcanol., v. 33, no. 3, p. 910–929.
- Tilling, R.I., 2008, The critical role of volcano monitoring in risk reduction: Adv. Geosci., v. 14, p. 3–11.
- United States Geological Survey, 1999, Exploring the deep ocean floor: Online, http://pubs.usgs.gov/gip/dynamic/exploring.html, accessed July 2016.
- United States Geological Survey, 2012, Black Rock Desert Volcanic Field: Online, http://volcanoes.usgs.gov/volcanoes/black_rock_desert/, accessed July 2016.
- USGS, 2001, Dual volcanic tragedies in the Caribbean led to founding of HVO: Online, http://hvo.wr.usgs.gov/volcanowatch/archive/2001/01_05_03.html, accessed July 2016.
- USGS, 2011, Volcanoes: Principal Types of Volcanoes: Online, http://pubs.usgs.gov/gip/volc/types.html, accessed July 2016.
- USGS, 2012a, USGS: Volcano Hazards Program: Online, https://volcanoes.usgs.gov/vhp/hazards.html, accessed July 2016.
- USGS, 2012b, Yellowstone Volcano Observatory: Online, https://volcanoes.usgs.gov/volcanoes/yellowstone/yellowstone_geo_hist_52.html, accessed July 2016.
- USGS, 2016, Volcanoes General – What are the different types of volcanoes? Online, https://www2.usgs.gov/faq/categories/9819/2730, accessed March 2017.
- USGS, 2017, The Volcanoes of Lewis and Clark – Mount St. Helens: Online, https://volcanoes.usgs.gov/observatories/cvo/Historical/LewisClark/Info/summary_mount_st_helens.shtml, accessed March 2017.
- Wallace, P.J., 2005, Volatiles in subduction zone magmas: concentrations and fluxes based on melt inclusion and volcanic gas data: Journal of Volcanology and Geothermal Research, v. 140, no. 1–3, p. 217–240., doi: 10.1016/j.jvolgeores.2004.07.023.
- Williams, H., 1942, The Geology of Crater Lake National Park, Oregon: With a Reconnaissance of the Cascade Range Southward to Mount Shasta: Carnegie institution.
Figure References
Figure 4.1: Lava flow in Hawai’i. Brocken Inaglory. 2007. CC BY-SA 3.0. https://commons.wikimedia.org/wiki/File:P%C4%81hoehoe_and_Aa_flows_at_Hawaii.jpg
Figure 4.2: Half Dome, an intrusive igneous batholith in Yosemite National Park. Jon Sullivan. 2004. Public domain. https://commons.wikimedia.org/wiki/File:Yosemite_20_bg_090404.jpg
Figure 4.3: Granite is a classic coarse-grained (phaneritic) intrusive igneous rock. James St. John. 2019. CC BY 2.0. https://commons.wikimedia.org/wiki/File:Granite_47_(49201189712).jpg
Figure 4.4: Basalt is a classic fine-grained extrusive igneous rock. James St. John. 2019. CC BY 2.0. https://commons.wikimedia.org/wiki/File:Basalt_3_(48674276863).jpg
Figure 4.5: Porphyritic texture. Jstuby. 2008. Public domain. https://commons.wikimedia.org/wiki/File:Olearyandesite.jpg
Figure 4.6: Pegmatitic texture. Jstuby. 2007. Public domain. https://commons.wikimedia.org/wiki/File:We-pegmatite.jpg
Figure 4.7: Scoria. Jonathan Zander (Digon3). 2008. CC BY-SA 3.0. https://commons.wikimedia.org/wiki/File:Scoria_Macro_Digon3.jpg
Figure 4.8: Pumice. deltalimatrieste. 2008. Public domain. https://commons.wikimedia.org/wiki/File:Pomice_di_veglia.jpg
Figure 4.9: Obsidian (volcanic glass). Note conchoidal fracture. Ji-Elle. 2011. CC BY-SA 3.0. https://commons.wikimedia.org/wiki/File:Lipari-Obsidienne_(5).jpg
Figure 4.10: Welded tuff. Wilson44691. 2010. Public domain. https://commons.wikimedia.org/wiki/File:HoleInTheWallTuff.JPG
Figure 4.11: Mineral composition of common igneous rocks. Woudloper. 2009. Public domain. https://commons.wikimedia.org/wiki/File:Mineralogy_igneous_rocks_EN.svg
Figure 4.12: Igneous rock classification table with composition as vertical columns and texture as horizontal rows. Kindred Grey. 2022. Adapted from Belinda Madsen, An Introduction to Geology. OpenStax. Salt Lake Community College. CC BY-NC-SA 4.0.
Table 4.1: Aphanitic and phaneritic rock types with images. Quartz monzonite 36mw1037 by B.W. Hallett, V. F. Paskevich, L.J. Poppe, S.G. Brand, and D.S. Blackwood via USGS (Public domain, https://commons.wikimedia.org/wiki/File:Quartz_monzonite_36mw1037.jpg). PinkRhyolite by Michael C. Rygel, 2014 (CC BY-SA 3.0, https://commons.wikimedia.org/wiki/File:PinkRhyolite.tif). Diorite MA by Amcyrus2012, 2015 (CC BY 4.0, https://commons.wikimedia.org/wiki/File:Diorite_MA.JPG). Andesite by James St. John, 2014 (CC BY 2.0, https://flic.kr/p/oBkKSy). GabbroRockCreek1 by Mark A. Wilson, 2008 (Public domain, https://commons.wikimedia.org/wiki/File:GabbroRockCreek1.jpg). VesicularBasalt1 by Jstuby, 2008 (Public domain, https://commons.wikimedia.org/wiki/File:VesicularBasalt1.jpg).
Figure 4.13: Dike of olivine gabbro cuts across Baffin Island in the Canadian Arctic. Mike Beauregard. 2012. CC BY 2.0. https://en.wikipedia.org/wiki/File:Franklin_dike_on_northwestern_Baffin_Island..jpg
Figure 4.14: Igneous sill intruding between Paleozoic strata in Nova Scotia. Mikenorton. 2010. CC BY-SA 3.0. https://commons.wikimedia.org/wiki/File:Horton_Bluff_mid-Carboniferous_sill.JPG
Figure 4.15: Quartz monzonite in the Cretaceous of Montana, USA. James St. John. 2010. CC BY 2.0. https://commons.wikimedia.org/wiki/File:Butte_Quartz_Monzonite_(Late_Cretaceous,_76_Ma;_Rampart_Mountain,_northeast_of_Butte,_Montana,_USA)_1.jpg
Figure 4.16: Half Dome in Yosemite National Park, California, is a part of the Sierra Nevada batholith which is mostly made of granite. Jon Sullivan. 2004. Public domain. https://commons.wikimedia.org/wiki/File:Yosemite_20_bg_090404.jpg
Figure 4.17: The Henry Mountains in Utah are interpreted to be a laccolith, exposed by erosion of the overlying layers. Steven Mahoney. 2005. CC BY-SA 2.5. https://commons.wikimedia.org/wiki/File:Henry_Mountains,_Utah,_2005-06-01.jpg
Figure 4.18: Laccolith forms as a blister in between sedimentary strata. Erimus and Stannered. 2007. Public domain. https://commons.wikimedia.org/wiki/File:Laccolith.svg
Figure 4.19: Bowen’s Reaction Series. Colivine. 2011. Public domain. https://commons.wikimedia.org/wiki/File:Bowen%27s_Reaction_Series.png
Figure 4.20: Olivine, the first mineral to crystallize in a melt. S kitahashi. 2006. CC BY-SA 3.0. https://commons.wikimedia.org/wiki/File:Peridot2.jpg
Figure 4.21: Norman L. Bowen. Unknown author. 1909. Public domain. https://commons.wikimedia.org/wiki/File:NormanLBowen_1909.jpg
Figure 4.22: Norman L. Bowen and his colleague working at the Carnegie Institution of Washington Geophysical Laboratory. Smithsonian Institution. 2010. Public domain. https://commons.wikimedia.org/wiki/File:(left_to_right)-_Norman_Levi_Bowen_(1887-1956)_and_Orville_Frank_Tuttle_(1916-1983)_(4730112454)_(cropped).jpg
Figure 4.23: Geothermal gradient. Bkilli1. 2013. CC BY-SA 3.0. https://commons.wikimedia.org/wiki/File:Temperature_schematic_of_inner_Earth.jpg
Figure 4.24: Pressure-temperature diagram showing temperature in degrees Celsius on the x-axis and depth below the surface in kilometers (km) on the y-axis. Kindred Grey. 2022. CC BY-SA 3.0. Adapted from Partial melting asthenosphere EN by Woudloper, 2010 (CC BY-SA 3.0, https://commons.wikimedia.org/wiki/File%3APartial_melting_asthenosphere_EN.svg).
Figure 4.25: Association of volcanoes with plate boundaries. Jose F. Vigil via USGS. 1997. Public domain. https://commons.wikimedia.org/wiki/File:Tectonic_plate_boundaries.png
Figure 4.26: Map of spreading ridges throughout the world. Eric Gaba. 2006. CC BY-SA 2.5. https://commons.wikimedia.org/wiki/File:Spreading_ridges_volcanoes_map-fr.svg
Figure 4.27: Pillow basalt on sea floor near Hawai’i. NOAA. 1988. Public domain. https://commons.wikimedia.org/wiki/File:Nur05018-Pillow_lavas_off_Hawaii.jpg
Figure 4.28: Black smoker hydrothermal vent with a colony of giant (6’+) tube worms. NOAA. 2006. Public domain. https://commons.wikimedia.org/wiki/File:Main_Endeavour_black_smoker.jpg
Figure 4.29: Distribution of hydrothermal vent fields. DeDuijn. 2016. CC BY-SA 4.0. https://commons.wikimedia.org/wiki/File:Distribution_of_hydrothermal_vent_fields.png
Figure 4.30: Distribution of volcanoes on the planet. USGS. 2007. Public domain. https://commons.wikimedia.org/wiki/File:Map_plate_tectonics_world.gif
Figure 4.31: Basaltic cinder cones of the Black Rock Desert near Beaver, Utah. Lee Siebert via Smithsonian Institution. 1996. Public domain. https://commons.wikimedia.org/wiki/File:Black_Rock_Desert_volcanic_field.jpg
Figure 4.32: Diagram showing a non-moving source of magma (mantle plume) and a moving overriding plate. Los688. 2008. Public domain. https://en.wikipedia.org/wiki/File:Hotspot(geology)-1.svg
Figure 4.33: The track of the Yellowstone hotspot, which shows the age of different eruptions in millions of years ago. Kelvin Case. 2013. CC BY 3.0. https://commons.wikimedia.org/wiki/File:HotspotsSRP_update2013.JPG
Figure 4.34: The Hawaiian–Emperor seamount and island chain. Ingo Wölbern. 2008. Public domain. https://commons.wikimedia.org/wiki/File:Hawaii-Emperor_engl.png
Figure 4.35: Mt. Shasta in Washington state with Shastina, its parasitic cone. Don Graham. 2013. CC BY-SA 2.0. https://commons.wikimedia.org/wiki/File:Mt._Shasta_and_Mt._Shastina,_CA_9-13_(26491330883).jpg
Figure 4.36: Oregon’s Crater Lake was formed about 7700 years ago after the eruption of Mount Mazama. Zainubrazvi. 2006. CC BY-SA 3.0. https://en.wikipedia.org/wiki/File:Crater_lake_oregon.jpg
Figure 4.37: Kilauea in Hawai’i. Quinn Dombrowski. 2007. CC BY-SA 2.0. https://commons.wikimedia.org/wiki/File:Kilauea_Shield_Volcano_Hawaii_20071209A.jpg
Figure 4.38: Eruption of Kilauea in 2018 produced high viscosity lava shown here crossing a road. USGS. 2018. Public domain. https://commons.wikimedia.org/wiki/File:USGS_K%C4%ABlauea_multimediaFile-1955.jpg
Figure 4.39: Olympus Mons, an enormous shield volcano on Mars, the largest volcano in the solar system, standing about two and a half times higher than Everest is above sea level. NASA. 1978. Public domain. https://en.wikipedia.org/wiki/File:Olympus_Mons_alt.jpg
Figure 4.40: Ropey pahoehoe lava. Bbb. 2010. GNU Free Documentation License 1.2. https://de.wikivoyage.org/wiki/Datei:ReU_PtFournaise_Lavastr%C3%B6me.jpg
Figure 4.41: Blocky a’a lava. Librex. 2009. CC BY 2.0. https://commons.wikimedia.org/wiki/File:Lava_del_Volcan_Pacaya_2009-11-28.jpg
Figure 4.42: Volcanic fissure and flow, which could eventually form a lava tube. NPS. 2004. Public domain. https://commons.wikimedia.org/wiki/File:Volcano_q.jpg
Figure 4.43: Devils Tower in Wyoming has columnar jointing. Colin.faulkingham. 2005. Public domain. https://commons.wikimedia.org/wiki/File:Devils_Tower_CROP.jpg
Figure 4.44: Columnar jointing on Giant’s Causeway in Ireland. Udri. 2014. CC BY-NC-SA 2.0. https://flic.kr/p/2j1mgwE
Figure 4.45: Mount Rainier towers over Tacoma, Washington. Lyn Topinka via USGS. 1984. Public domain. https://commons.wikimedia.org/wiki/File:Mount_Rainier_over_Tacoma.jpg
Figure 4.46: Mt. Fuji in Japan, a typical stratovolcano, symmetrical, increasing slope, visible crater at the top. Alpsdake. 2016. CC BY-SA 4.0. https://commons.wikimedia.org/wiki/File:Numazu_and_Mount_Fuji.jpg
Figure 4.47: Lava domes have started the rebuilding process at Mount St. Helens, Washington. Willie Scott via USGS. 2006. Public domain. https://commons.wikimedia.org/wiki/File:MSH06_aerial_crater_from_north_high_angle_09-12-06.jpg
Figure 4.48: Timeline of events at Mount Mazama. USGS and NPS. 2006. Public domain. https://commons.wikimedia.org/wiki/File:Mount_Mazama_eruption_timeline.PNG
Figure 4.49: Wizard Island sits in the caldera at Crater Lake. Don Graham. 2006. CC BY-SA 2.0. https://commons.wikimedia.org/wiki/File:Crater_Lake_National_Park,_OR_2006_(6539577313).jpg
Figure 4.50: Map of calderas and related rocks around Yellowstone. USGS. 1905. Public domain. https://www.usgs.gov/media/images/simplified-map-yellowstone-caldera
Figure 4.51: Several prominent ash beds found in North America, including three Yellowstone eruptions shaded pink (Mesa Falls, Huckleberry Ridge, and Lava Creek), the Bisho Tuff ash bed (brown dashed line), and the modern May 18th, 1980 ash fall (yellow). USGS. 2005. Public domain. https://commons.wikimedia.org/wiki/File:Yellowstone_volcano_-_ash_beds.svg
Figure 4.52: Sunset Crater, Arizona is a cinder cone. NPS. Unknown date. Public domain. https://commons.wikimedia.org/wiki/File:Sunset_Crater10.jpg
Figure 4.53: Soon after the birth of Parícutin in 1943. K. Segerstrom via USGS. 1943. Public domain. https://commons.wikimedia.org/wiki/File:Paricutin_30_612.jpg
Figure 4.54: Lava from Parícutin covered the local church and destroyed the town of San Juan, Mexico. Sparksmex. 2007. Public domain. https://commons.wikimedia.org/wiki/File:Paricutin2.jpg
Figure 4.55: World map of flood basalts. Williamborg. 2011. CC BY-SA 3.0. https://commons.wikimedia.org/wiki/File:Flood_Basalt_Map.jpg
Figure 4.56: Igneous rock types and related volcano types. Unknown author. Unknown date. “Personal use.” https://www.seekpng.com/ipng/u2t4y3r5o0r5r5w7_table-of-igneous-rocks-and-related-volcano-types/
Figure 4.57: General diagram of volcanic hazards. USGS. 2008. Public domain. https://pubs.usgs.gov/fs/fs002-97/old%20html%20files/
Figure 4.58: Human remains from the 79 CE eruption of Vesuvius. Gary Todd. 2019. Public domain. https://commons.wikimedia.org/wiki/File:Pompeii_Ruins_Cast_of_Human_Victim_at_Villa_of_the_Mysteries_(48445486616).jpg
Figure 4.59: Mount St. Helens, the day before the May 18th, 1980 eruption (left) and 4 months after the major eruption (right). Mount St. Helens, one day before the devastating eruption by Harry Glicken, USGS/CVO, 1980 (Public domain, https://commons.wikimedia.org/wiki/File:Mount_St._Helens,_one_day_before_the_devastating_eruption.jpg). MSH80 st helens from johnston ridge 09-10-80 by Harry Glicken via USGS (Public domain, https://commons.wikimedia.org/wiki/File:MSH80_st_helens_from_johnston_ridge_09-10-80.jpg).
Figure 4.60: Image from the May 18, 1980, eruption of Mt. Saint Helens, Washington. Austin Post via USGS. 1980. Public domain. https://commons.wikimedia.org/wiki/File:MSH80_eruption_mount_st_helens_05-18-80-dramatic-edit.jpg
Figure 4.61: The material coming down from the eruption column is a pyroclastic flow. C.G. Newhall via USGS. 1984. Public domain. https://en.wikipedia.org/wiki/File:Pyroclastic_flows_at_Mayon_Volcano.jpg#:~:text=English%3A%20Pyroclastic%20flows%20at%20Mayon,50%20km%20toward%20the%20west.
Figure 4.62: The remains of St. Pierre. Angelo Heilprin. 1902. Public domain. https://commons.wikimedia.org/wiki/File:Pelee_1902_3.jpg
Figure 4.63: Sequence of events for Mount St. Helens, May 18th, 1980. Lyn Topinka via USGS. 1998. Public domain. https://commons.wikimedia.org/wiki/File:Msh_may18_sequence.gif
Figure 4.64: Aman sweeps ash from an eruption of Kelud, Indonesia. Crisco 1492. 2014. CC BY-SA 3.0. https://commons.wikimedia.org/wiki/File:Ash_in_Yogyakarta_during_the_2014_eruption_of_Kelud_01.jpg
Figure 4.65: Micrograph of silica particle in volcanic ash. USGS. 1980. Public domain. https://volcanoes.usgs.gov/volcanic_ash/components_ash.html
Figure 4.66: Mud line shows the extent of lahars around Mount St. Helens. USGS. 1980. Public domain. https://www.usgs.gov/media/images/lahars-resulting-may-18-1980-eruption-mount-st-helens
Figure 4.67: Old lahars around Tacoma, Washington. USGS. 1905. Public domain. https://www.usgs.gov/media/images/lahar-pathways-events-heading-mount-rainier-map-showing-t
Liquid rock within the Earth.
The theory that the outer layer of the Earth (the lithosphere) is broken in several plates, and these plates move relative to one another, causing the major topographic features of Earth (e.g. mountains, oceans) and most earthquakes and volcanoes.
A series of mineral formation temperatures that can explain the minerals that form in specific igneous rocks. For example, pyroxene will form with olivine and amphibole, but not quartz.
A natural substance that is typically solid, has a crystalline structure, and is typically formed by inorganic processes. Minerals are the building blocks of most rocks.
The process of liquid rock solidifying into solid rock. Because liquid rock is made of many components, the process is complex as different components solidify at different temperatures.
Rocks that are formed from liquid rock, i.e. from volcanic processes.
The resistance of a fluid to flow, where a high value means a fluid which does not like to flow (like toothpaste), and a low value means a fluid which flows easily (like water).
Place where lava is erupted at the surface.
The mineral makeup of a rock, i.e. which minerals are found within a rock.
A solid part of the lithosphere which moves as a unit, i.e. the entire plate generally moves the same direction at the same speed.
Liquid rock on the surface of the Earth.
The outer physical layer of the core, which is liquid. Movement within the outer core is believed to be responsible for Earth's magnetic field and flips of the magnetic field.
Middle chemical layer of the Earth, made of mainly iron and magnesium silicates. It is generally denser than the crust (except for older oceanic crust) and less dense than the core.
The outermost chemical layer of the Earth, defined by its low density and higher concentrations of lighter elements. The crust has two types: continental, which is the thick, more ductile, and lowest density, and oceanic, which is higher density, more brittle, and thinner.
Igneous rock cooling, and thus forming, outside of the Earth, i.e. on the surface.
An extrusive rock filled with small bubble structures, frozen in place as gases escaped from the cooling lava.
Igneous rock cooling, and thus forming, inside of the Earth, i.e. under the surface.
Arrangement of minerals within a rock.
Large, easy-to-see crystals within an igneous rock. This is common in intrusive rocks.
Small, microscopic, hard-to-see crystals (i.e. no visible crystals) within an igneous rock. This is common in extrusive rocks.
Volcanic tephra that is less than 2 mm in diameter.
Dark colored volcanic glass, with extremely small microscopic crystals or no crystals. Typically form from felsic volcanism.
An igneous rock with two distinctive crystal sizes.
A large crystal within an igneous rock. These can be seen within phaneritic and porphyritic rocks.
General term for the fine-grained, not discernible part of a rock. In igneous rocks, this is the part of the rock that is not phenocrysts, and can help in determining the composition of extrusive rocks. In sedimentary rocks, it typically refers to the fine-grained components, namely mud. In metamorphic rocks, it is usually referring to material between porphyroblasts or a low-grade rock with only microscopic mineralization.
Consisting of three end members: potassium feldspar (K-spar, KAlSi3O8), plagioclase with calcium (CaAl2Si2O8, called anorthite), and plagioclase with sodium (NaAlSi3O8, called albite). Commonly blocky, with two cleavages at ~90°. Plagioclase is typically more dull white and gray, and K-spar is more vibrant white, orange, or red.
SiO2. Transparent, but can be any color imaginable with impurities. No cleavage, hard, and commonly forms equant masses. Perfect crystals are hexagonal prisms topped with pyramidal shapes. One of the most common minerals, and is found in many different geologic settings, including the dominant component of sand on the surface of Earth. Structure is a three-dimensional network of silica tetrahedra, connected as much as possible to each other.
X1A2-3Z4O10(OH, F)2, where commonly X=K, Na, Ca; A=Al, Mg, Fe; Z=Si, Al. Has two more-common occurrences, light-colored (translucent and pearly tan) muscovite, and dark-colored biotite. Has one strong cleavage, and is typically seen as sheets, in stacks or "books." Common in many igneous and metamorphic rocks. Structure is two-dimensional sheets of silica tetrahedra in a hexagonal network.
A rock (or texture within a rock) with unusually-large crystals, minerals with rare trace element concentrations, and/or unusual minerals, typically forming in veins as the last dredges of magma crystallize.
A weakness within the atomic structure of a mineral, which allows the mineral to break more easily along that plane. Minerals can have one, two, three, or more cleavages. Cleavage can also refer to the alignment of features within metamorphic rocks., though they are unrelated.
The process in which solids (like minerals) are disassociated and the ionic components are dispersed in a liquid (usually water).
Components of magma which are dissolved until it reaches the surface, where they expand. Examples include water and carbon dioxide. Volatiles also cause flux melting in the mantle, causing volcanism.
The act of taking a solid and dissolving it into a liquid. This commonly occurs with salts and other minerals in water.
Low density, highly vesiculated, usually white to tan volcanic rock. Typically arises from felsic volcanism.
Fractures that have a circular appearance.
A break within a rock that has no relative movement between the sides. Caused by cooling, pressure release, tectonic forces, etc.
The gases that are part of the Earth, which are mainly nitrogen and oxygen.
General term for solid, but fragmented, material erupted from a volcano. Has three subcomponents: ash (<2mm), lapilli (2-64 mm), blocks and bombs (>64mm).
Rocks (or rock textures) that are formed from explosive volcanism.
Sedimentary rocks that are made of sediment, weathered pieces of bedrock.
Volcanic tephra that has a diameter between 2 mm and 64 mm. Many cinders are within the category of lapilli.
Large volcanic tephra greater than 64 mm in diameter.
Rocks made from pyroclastic tephra: either ash, lapilli, and/or bombs. Tephra type can be used as an adjective, i.e. ash-fall tuff. If deposited hot, where material can fuse together while hot, the rock is then called a welded tuff.
Can refer to a volcanic rock with higher silica composition, or the minerals that make up those rocks, namely quartz, feldspar, and muscovite mica. Felsic rocks are lighter in color and contain more minerals that are light in color. Primary felsic rocks are rhyolite (extrusive) and granite (intrusive).
A volcanic rock with medium silica composition, equally rich in felsic minerals (feldspar) and mafic minerals (amphibole, biotite, pyroxene). Intermediate rocks are grey in color and contain somewhat equal amounts of minerals that are light and dark in color. Primary intermediate rocks are andesite (extrusive) and diorite (intrusive).
Can refer to a volcanic rock with lower silica composition, or the minerals that make up those rocks, namely olivine, pyroxene, amphibole, and biotite. Mafic rocks are darker in color and contain more minerals that are dark in color, but can contain some plagioclase feldspar. Primary mafic rocks are basalt (extrusive) and gabbro (intrusive).
An igneous rock with extremely low silica composition, being made of almost all olivine and pyroxene. Ultramafic rocks contain very low amount of silica and are common in the mantle. Primary ultramafic rocks are komatiite (extrusive) and peridotite (intrusive).
General name of a felsic rock that is intrusive. Has more felsic minerals than mafic minerals.
A group of chain silicate minerals that form needlelike or prismatic crystals. Can be many colors but the most common form, hornblende, is dark brown to black. Has oblique cleavages at 54° and 126°. Common in many igneous rocks and some metamorphic rocks.
XY(Al,Si)2O6, in which X typically equals Na, Ca, Mg, or Fe and Y typically equals Mg, Fe, or Al. Typically black to dark green, blocky, with two cleavages at ~90°. Common in mafic igneous rocks and some metamorphic rocks. Structure is a single chain of silica tetrahedra.
(Fe,Mg)2SiO4. Typically translucent olive green and equant, with no cleavage. Common in mafic igneous rocks and in the mantle, but easily weathered in surface conditions. Structure is isolated silica tetrahedra. Known as peridot when a gem.
An intrusive ultramafic rock, which is the main component of the mantle. The minerals in peridotite are typically olivine with some pyroxene.
General name of a felsic rock that is extrusive. Generally has a white, tan, or pink groundmass color.
General name of an intermediate igneous rock that is extrusive. Generally has a gray groundmass color.
General name of an intermediate rock that is intrusive. Has about the same amount of felsic minerals and mafic minerals.
General name of a mafic rock that is extrusive. Generally has a black groundmass color.
General name of a mafic rock that is intrusive. Has more mafic minerals than felsic minerals.
Eon defined as the time between 4 billion years ago to 2.5 billion years ago. Most of the oldest rocks on Earth, including large portions of the continents, formed at this time.
A group of all atoms with a specific number of protons, having specific, universal, and unique properties.
The transport and movement of weathered sediments.
Initiation point of an earthquake or fault movement.
Term for the underlying lithified rocks that make up the geologic record in an area. This term can sometimes refer to only the deeper, crystalline (non-layered) rocks.
A narrow igneous intrusion that cuts through existing rock, not along bedding planes.
A sheet-like igneous intrusion that has intruded parallel to bedding planes within the bedrock.
Discernible layers of rock, typically from a sedimentary rock.
A reservoir of magma below a volcano.
Rocks which allow petroleum resources to collect or move.
A ductile material that moves toward the surface of Earth. Can be used to describe salt domes and intrusions.
Minerals made from just a single element, bonded to itself. Examples include gold, silver, copper, and diamond, which is a native version of carbon.
An accepted scientific idea that explains a process using the best available information.
The process of surrounding bedrock being broken off and passed through a magma.
A coherent body of intrusive rock (which formed underground) which is now at (or near) the surface.
Used to describe a large mass or chain of many plutons and intrusive rocks.
An extensive, distinct, and mapped set of geologic layers.
A process where an oceanic plate descends bellow a less dense plate, causing the removal of the plate from the surface. Subduction causes the largest earthquakes possible, as the subducting plate can lock as it goes down. Volcanism is also caused as the plate releases volatiles into the mantle, causing melting.
Large igneous intrusion that is wedged between sedimentary layers, bulging upwards. Called a lopolith if bulging downward.
The measure of the vibrational (kinetic) energy of a substance.
A proposed explanation for an observation that can be tested.
A unit of the geologic time scale; smaller than an era, larger than an epoch. We are currently in the Quaternary period.
The study of rocks, either macroscopically or microscopically. This study is typically divided into one of the three rock types (e.g. igneous petrology).
The process of atoms breaking down randomly and spontaneously.
The average change in temperature that is experienced as material moves into the Earth. Near the surface, this rate is about 25°C/km.
The innermost chemical layer of the Earth, made chiefly of iron and nickel. It has both liquid and solid components.
Melting that occurs as material is moved upward and pressure is released, typically found at divergent plate boundaries or hot spots.
The process in which volatiles enter the mantle wedge, and the volatiles lower the melting temperature, causing volcanism.
The process of some material being derived from a heterogenous mixture when melting (e.g. rocks). Because all rocks are made of many different components, they have many different melting points. As they are heated, certain easy-to-melt components will be melted first.
Activity that occurs at the boundaries between plates.
A divergent boundary within an oceanic plate, where new lithosphere and crust is created as the two plates spread apart. Mid-ocean ridge and spreading center are synonyms.
The layers of igneous, sedimentary, and metamorphic rocks that form the continents. Continental crust is much thicker than oceanic crust. Continental crust is defined as having higher concentrations of very light elements like K, Na, and Ca, and is the lowest density rocky layer of Earth. Its average composition is similar to granite.
Area of extended continental lithosphere, forming a depression. Rifts can be narrow (focused in one place) or broad (spread out over a large area with many faults).
Activities that occur within plates, away from plate boundaries.
Rising stationary magma, forming a succession of volcanism. This is reflected as islands on oceanic plates, and volcanic mountains or craters on land.
Relatively flat ocean floor, which accumulates very fine grained detrital and chemical sediments.
Place where two plates are moving apart, creating either a rift (continental lithosphere) or a mid-ocean ridge (oceanic lithosphere).
Location where two plates are in contact, allowing a relative motion between the two plates. These are the locations where most earthquakes and volcanoes are found.
The thin, outer layer of the Earth which makes up the rocky bottom of the ocean basins. Oceanic crust is much thinner (but denser) than continental crust. Oceanic crust is made of rocks similar to basalt and as it cools, becomes more dense.
The outermost physical layer of the Earth, made of the entire crust and upper mantle. It is brittle and broken into a series of plates, and these plates move in various ways (relative to one another), causing the features of the theory of plate tectonics.
Metamorphism which occurs with hot fluids going within rocks, altering and changing the rocks.
Mineral chimneys that form at hydrothermal vents.
A biologic process of gaining energy from chemicals from within the Earth, similar to using the energy of the sun in photosynthesis.
Minerals bonded via a sulfur (S-2) atom.
Place where two plates come together, casing subduction or collision.
Name given to the subducting plate, where volatiles are driven out at depth, causing volcanism.
Place with a chain of mountain volcanism on a continent, from oceanic-continental subduction.
Term for the extensional tectonic province that extends from California’s Sierra Nevada Mountains in the west, to Utah’s Wasatch Mountains to the east, to southern Oregon and Idaho to the north, to northern Mexico to the south. Known as a wide rift, each graben (basin) is bounded by horsts (ranges).
Rare very low viscosity eruption that covers vast areas. None have been observed in human history.
A type of tephra which forms as blobs of magma splatter out of a volcanic vent (e.g. cinder cone) and cool and harden quickly.
Stresses that pull objects apart into a larger surface area or volume; stretching forces.
Rising material and heat derived from the mantle. These may be responsible for hot spots.
When a species no longer exists.
Volcano with steep sides, made of a composite of many types of eruption styles, from low viscosity mafic magma, higher viscosity felsic lava, but most commonly, intermediate andesite lava.
Pipe that connects the magma chamber to the volcanic vent.
Opening of a volcano where lava can erupt.
Small side vent of a stratovolcano where secondary eruption can occur.
Hole left behind after a large volume of material erupts out of a volcano. This depression is often turned into a valley or lake after the eruption is over.
An exposed part of a craton.
Volcano with a gentle slope, formed from low viscosity, low volatile, mafic, basaltic lava.
to move in a circular or curving course or orbit. Not to be confused with rotate, when something spins on an axis
Rope-like, flowing basaltic lava.
A blocky, stubby, rubble-like lava.
Energy that radiates from fault movement via earthquakes.
A rock up-warping of symmetrical anticlines.
A down-warped feature in the crust.
Very steep sided volcanic feature formed by higher viscosity, higher-silica lava.
An interconnected set of parts that combine and make up a whole.
A period of cooler temperatures on Earth in which ice sheets can grow on continents.
A channelled body of water.
Volcano formed from piles of cinders and tephra. Forms with low viscosity lava with high volatile content.
A pronounced increase in the extinction rate, typically caused by significant environmental change. There have been 5 mass extinctions in geologic history, and a sixth that has been suggested to be currently occurring.
General term for sudden material falling (sliding) down a slope due to gravity.
A collapsed part of the eruption column that travels down at very hot temperatures and very fast speeds. They are the most dangerous immediate volcanic hazard.
Material filling in a cavity left by a organism that has dissolved away.
Water that is below the surface.
A series of waves produced from a sudden movement of the floor of a ocean basin (or large lake), caused by events such as earthquakes, volcanic eruptions, landslides, and bolide impacts.
Detached, free-falling rocks from very steep slopes.
Non-directional pressure resulting from burial.
Minerals based on bonds to column 17 halogens, such as chlorine and fluorine.
A geologic circumstance (such as a fold, fault, change in lithology, etc.) which allows petroleum resources to collect.
An event that causes a landslide event. Water is a common trigger.
A feature with no internal structure, habit, or layering.
A type of volcanic mudslide, in which rain or snowmelt accumulates volcanic ash of the slopes of steep volcanoes or other mountains and then wash downhill, causing damaging flooding.
A body of ice that moves downhill under its own mass.
The process of changing a magma's composition, usually through assimilation or fractionation.
Bedrock around the magma chamber being incorporated into the magma, sometimes changing the composition of the magma.
When two continents crash, with no subduction (and thus little to no volcanism), since each continent is too buoyant. Many of the largest mountain ranges and broadest zones of seismic activity come from collisions.