Chapter 5: Abiotic Stress Effects on Plant Growth and Development
Chapter Contents
This chapter is devoted to understanding the effects of abiotic stresses (chemical and physical) on the growth and development of plants. Crop losses due to abiotic stress is considered to be the principal cause of crop yield loss worldwide. Economic losses are in the billions of dollars and with increased global temperatures, extreme weather events, and climate change, it is increasingly important to have an understanding of how abiotic stress affects plant growth and distribution. Not only are commercial crops impacted by abiotic stresses but landscapes, home gardens and natural ecosystems are also affected. The goal of this chapter is to provide a framework for understanding how plants respond to abiotic stress. This chapter covers the basics of this topic. There are a number of other resources and research out there on this topic for those interested in taking a deeper dive.
Definition of Plant Stress
One way of defining stress is any change in environmental conditions that adversely affects survival, growth, development and yield in plants. Stress can be divided into two different types, biotic and abiotic. Biotic stresses are caused by another living organism, whereas, abiotic stresses are caused by the physical/chemical environment. In this chapter we are going to be concerned with the abiotic stresses and their impact on plant growth and development.
Abiotic Stress
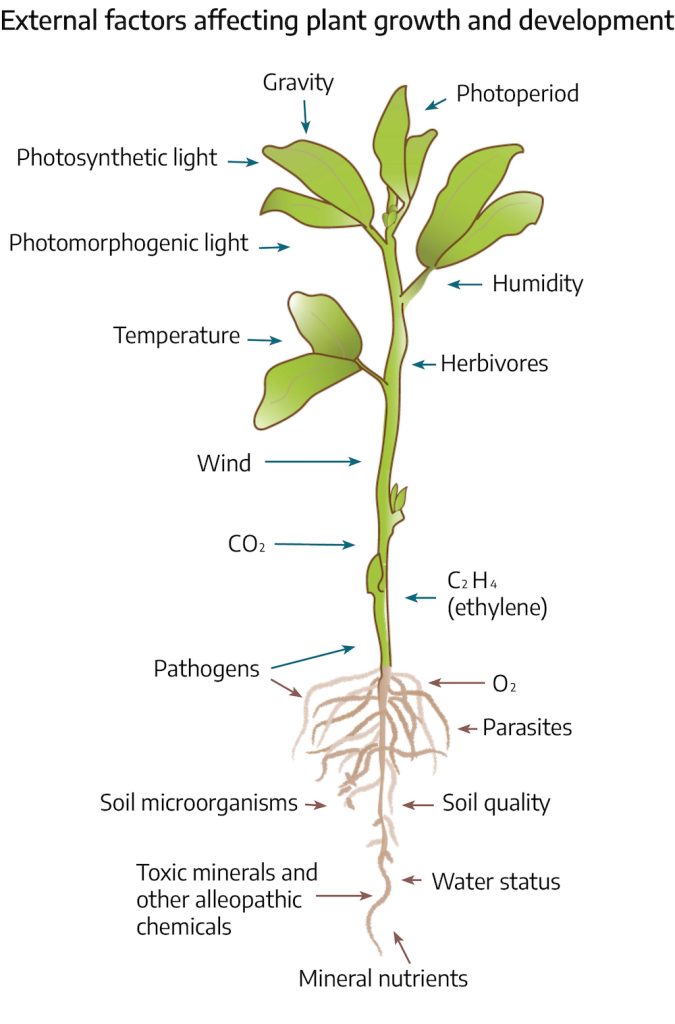
Abiotic stress can divide into physical and chemical stresses. Physical stresses include: water (drought/flooding), temperature (high/low), light (quantity/quality), and mechanical (ice/snow/wind). Chemical stresses include nutrient (high/low), salinity, soil characteristics (pH/soil composition), air pollution (CO2/O2/NO2/SO2) and pesticides (herbicides/insecticides/fungicides). Each of these types of stress will be discussed in this chapter.
Plant Responses to Abiotic Stress
Plant responses to stress depend on the severity, duration, number of exposures and if a single stress or multiple stresses are involved. Rarely are plants in nature exposed to a single stress. For example, if a plant is exposed to freezing temperatures, the duration and frequency of exposure may be critical. If the temperature is low enough to freeze the soil in which the plant is growing, the plant may suffer from water deficit as well as nutrient deficiency.
Plant part, stage of development and genetics are all plant characteristics that can determine susceptibility to stress. Using freezing temperatures as an example, young actively growing tissues are more susceptible to freezing than old, slower growing tissues. Flowering in fruit trees may be reduced during freezing temperatures resulting in failure of fruits to develop. Some plants such as spinach and peas are genetically more resistant to cold than are tomato plants.
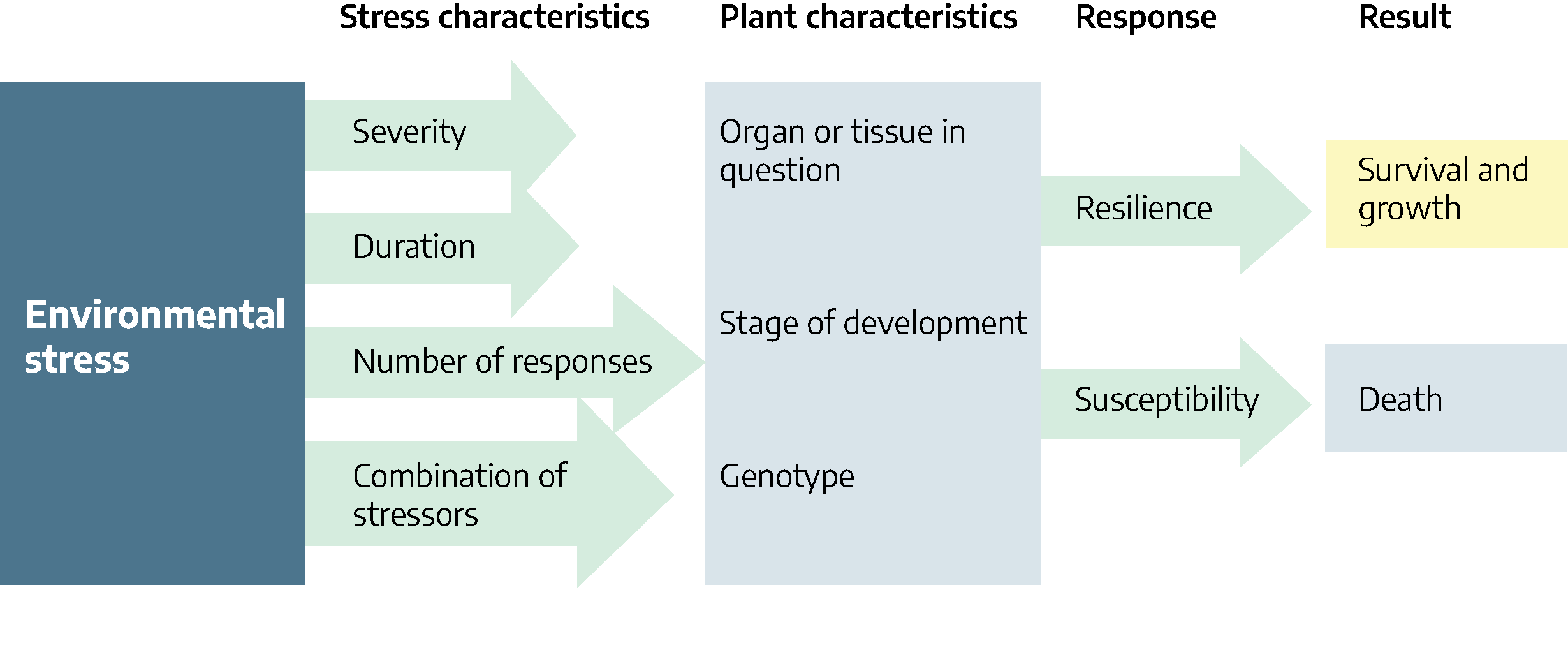
Chemical Stress
Air Pollution Stress
There are many chemicals that comprise what is referred to as air pollution. Such chemicals include: carbon dioxide, ozone, sulphur dioxide, hydrogen fluoride, oxides of nitrogen, olefins such as ethylene (a natural plant hormone), ammonia, chlorine, hydrogen chloride and metals. The burning of fossil fuels, natural processes, industrial activities, and atmospheric photochemical activity can produce air pollution. In this section only carbon dioxide, ozone, nitrous oxide, sulphur dioxide and ozone will be discussed. These are produced directly or indirectly from the combustion of fossil fuels.
Carbon dioxide pollution
Burning of fossil fuels, or any carbon-containing substance, produces carbon dioxide (CO2) as a result of combining carbon with atmospheric O2 under high temperature.
Increasing CO2 concentrations can influence plant growth directly through its effects on photosynthesis. As a greenhouse gas, it also affects plant growth by impacting atmospheric CO2 levels, which could be a contributor to global warming and global climate change. Atmospheric CO2 levels have increased from about 315 ppm in the mid 1950s to 412.5 ppm in 2020. Plants use CO2 , sunlight and water in photosynthesis to produce sugars that are used as an energy source to make other constituents necessary for plant growth and development.
Figure 5-3 illustrates how CO2 levels have changed over the past 800,000 years compared to today’s levels and Figure 5-4 shows how CO2 levels have changed since 1961.
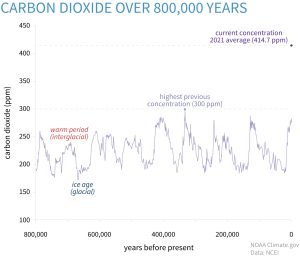
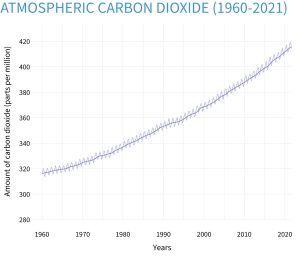
Effects of increased CO2 levels on plant growth, development, and distribution
All plants are not the same with respect to how they will respond to rising CO2 levels. These differences have to do with how a plant captures (fixes) CO2 from the air and processes it into sugar.
Plants can be divided into 3 groups based on how they remove CO2 from the atmosphere. These are referred to as C3, C4, and CAM plants. Each plant type has adapted to a different environmental niche and has a different way to fix CO2 from the atmosphere.
Most C3 plants are found in temperate to cold climates with high moisture environments and represent the majority of plant species. They typically are slower-growing plants than C4 plants and use CO2 less efficiently as a result of an energy-wasting process called photorespiration. There are 250,000 known C3 species (1854 weed species). Examples of C3 plants include: forest trees, woody shrubs, cool season grasses, soybean, wheat and most vegetable crops.
C4 plants are found in hot, dry, subtropical to tropical environments. They are fast growers and have higher rates of photosynthesis than C3 plants. Because C4 plants do not photorespire, they are more efficient in fixing CO2 than C3 plants, and thus when growing under high light and limited water conditions, C4 plants can outperform C3 plants. There are 7500 known C4 species (146 weed species). Corn, crab grass, millet, sorghum, sedge, nut grass and sugar cane are considered C4 plants. Interestingly, although C4 plants comprise only about 5% of the world’s biomass, they fix roughly 30% of the terrestrial CO2.
CAM plants are found in very hot dry desert areas. Like C4 plants, CAM plants are not susceptible to photorespiration because, unlike C3 and C4 plants, they open their stomata at night, thus conserving water and fixing CO2 and storing it for use during the day to make sugar. There are 16,000 known CAM species. Pineapple, yucca, cactus, orchids, and jade plants are CAM plants.
The types of environments occupied by C3, C4 and CAM plants can be observed in the United States as one travels from the east coast to the west. The eastern deciduous forests (mostly C3 plants) start in the east and end near the border of Oklahoma, followed by the grasslands (mostly C4 plants) of the prairie states and into the deserts (CAM plants) of the far west.
Possible responses of C3, C4, and CAM plants to increasing temperature and elevated CO2
Experiments have shown that C3 and C4 plants grown at elevated CO2 levels grow faster. However, the growth response is usually greater in C3 plants because they are considered to be CO2-limited compared to C4 plants. In some cases, increased growth in C3 plants has been shown to be temporary, and some C4 plants do not respond to elevated CO2 levels at all. A recent long term 15-year field experiment using C3 and C4 grasses demonstrated that elevated CO2 levels may not affect C3 and C4 plants as previously predicted using short-term studies. The authors found elevated CO2 caused a slight increase in biomass of C3 plants over time, but the total yield declined for both elevated CO2 plants and controls (ambient CO2) until about year 10, when no further decline occurred and yield was about the same. In C4 grasses, little difference in yield occurred between elevated CO2 plants and ambient plants, but after 10 years, ambient CO2 plants had increased yields and elevated CO2-treated plants had greater yield than controls (Reich 2018). But photosynthesis also depends on temperature and the amount of light and water available. Since C4 plants have greater water use efficiency and are more heat tolerant than C3 plants, it is possible that as temperature increases and water availability declines, C4 plants may have an advantage over C3 plants. In other words there may be a cross-over point where higher temperature and lower water availability favor C4 plants over C3 plants even though C3 plants may benefit more from elevated CO2 levels.
Although not as much has been done with CAM plants relative to how climate change will affect these plants, it appears that CAM plants will benefit from elevated CO2 levels as well. On average, when CO2 levels are doubled, the biomass of CAM plants has been observed to increase as much as 35%. This suggests that this group of plants may do well as temperature and CO2 levels increase and occupy an expanded environmental niche in which C3 and C4 plants cannot exist.
All of this has ramifications for plant competition in natural ecosystems as well as in agro-ecosystems. As global warming increases, natural plant communities are shifting toward northern latitudes or alpine environments. Agricultural production areas and types of plants that grow in specific regions may have to be adjusted to accommodate climate change, with the possibility that entire regions once productive agriculturally may cease to be productive, and competition from weeds may produce new challenges with respect to control. Recently, USDA issued new growth zone designations for the United States in which climate zones have been pushed toward northern latitudes.
Nitrogen oxides, sulphur dioxide, and ozone pollution
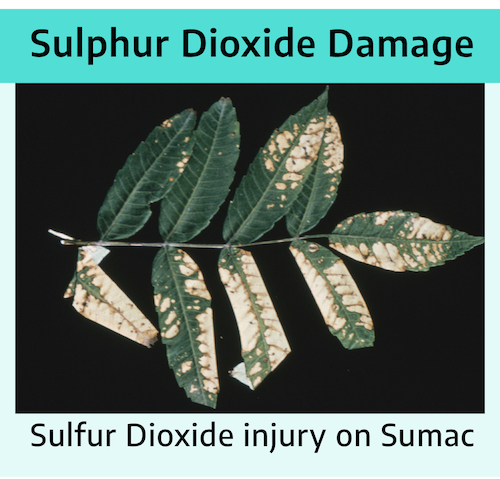
Oxides of carbon ( CO2 and CO), sulfur (SO2) and nitrogen (NO and NO2) are considered to be primary air pollutants because they come directly from a source such as automobile exhausts, burning of coal, refuse, or natural sources such as vegetation fires and volcanic activity. In the air, some of these pollutants (SO2 & NO2) combine with water to form acids and can fall as acid precipitation, or nitrogen oxides can undergo photochemical reactions to produce ozone (O3). These are considered to be secondary pollutants formed from the primary pollutants.
Effects of acid rain from nitrogen oxides and sulfur oxides on plant growth
Considerable research has been conducted on acid rain effects on forest trees. Results suggest that acid rain can impact forest ecosystems by leaching nutrients from leaves and/or affecting soil pH by making it more acidic. This in turn affects nutrient availability either directly or through leaching of nutrients out of the soil. Also, at these lower soil pH levels, aluminum in soils is more readily available to plants and may be at toxic levels affecting tree growth. As a result, this weakens trees and makes them more susceptible to disease, insects, cold, drought and other air pollutants such as O3 (ozone). There may be a more direct effect on trees that grow in elevated areas where they may be exposed to acid fog/clouds, resulting in longer exposure of leaves to acid conditions.
Agricultural crops are not as drastically affected by acid rain as are plants in natural ecosystems. One reason for this is that a grower can control soil pH and nutrient levels as needed.
The good news about atmospheric levels of nitrogen oxides and sulfur oxides is they have declined since 1980. EPA estimates indicate that emissions in 1980 of nitrogen oxides and sulfur oxides were 26 and 27 million tons, respectively, while in 2013 they were 5 and 13 million tons, respectively.
O3 effects on plant growth
Ozone is a strong oxidizing agent. It enters the plant through stomata during the day. It impacts plants by oxidizing and degrading membrane lipids and proteins that control permeability and biochemical reactions. Photosynthesis is very susceptible to O3 and is part of the reason reductions in growth and yield have been observed in crop plants. Dicots appear to be more susceptible to O3 than monocots. This may be because many monocots are C4 plants and more efficient than C3 plants at fixing CO2 and conserving water under high temperature dry conditions. USDA has determined that soybean yields can be reduced by 10% at O3 levels of 50 ppb, amounting to $1 billion in losses per year just from that single crop. Peanuts and cotton show reductions of 11% and 8%, respectively, at 50 ppb O3. Sorghum and field corn, both C4 plants, show little to no reductions in yield at that concentration. From 1980 to 2013, O3 levels have dropped by 33% but are still high enough to cause significant damage to natural and agricultural vegetation.
The following links are good references for more information on O3 and its effects on crop plants: “Ozone Research and Vegetative Impacts” by Ray Knighton, USDA CSREES, 2006, and “National Air Quality: Status and Trends of Key Air Pollutants,” US EPA website.
Plant Nutrient Stress
Nutritional stress in plants can be caused by either high or low concentrations of essential elements in the soil. It is important to have the soil analyzed for the concentrations of essential elements and also to determine the pH of the soil before planting (see Chapter 2 “Soils” for more information on soil testing). This will prevent addition of too much or too little fertilizer if the soil needs amending. Determining pH is important because soils may have plenty of nutrients that are simply are not available because extremes in pH can influence their availability to plants.
Essential Elements Needed for Plant Growth
Plants generally require thirteen elements, which they absorb as inorganic ions. This is in addition to carbon, hydrogen and oxygen, which they obtain from carbon dioxide, water and molecular oxygen. Six of these elements are required in greater amounts than the others and are called “macro-nutrients” or “major” elements. They are nitrogen, phosphorus, potassium, sulfur, calcium, and magnesium. The seven “micro-nutrients,” “minor,” or “trace” elements are iron, manganese, boron, copper, zinc, chlorine, and molybdenum. Several elements are required by some species but not by others. For example, sodium is required for certain blue-green algae and the halophyte Atriplex vescicaria. Cobalt is a micro-nutrient for some microorganisms and symbionts, although it has not been demonstrated to be essential for green plants. Silicon is indispensable for diatoms, and vanadium is reported to be essential for the green alga Scenedesmus obliquus. Twelve of the thirteen elements in Table 5-2 are derived from parent rock and are, therefore, “mineral elements.” The ultimate source of nitrogen is molecular nitrogen (N2) of the earth’s atmosphere. However, aside from those plants that fix atmospheric nitrogen (blue-green algae/bacteria either alone or symbiotically (nitrogen fixing bacteria on legume roots), nitrogen is absorbed as an inorganic ion (nitrate or ammonium). If any one of these elements is missing, plants will not grow and reproduce. For more detailed information about the role of essential elements in plants, see Chapter 1 “Botany.”
Table 5-1: Essential micronutrient elements and form absorbed by most plants
Element | Symbol | Chemical form most frequently absorbed | Conc. in Dry Tissue (ppm) |
---|---|---|---|
Molybdenum | Mo | MoO4 | 0.1 |
Copper | Cu | Cu+ Cu++ | 6 |
Zinc | Zn | Zn++ | 20 |
Manganese | Mn | Mn++ | 50 |
Iron | Fe | Fe++ Fe+++ | 100 |
Boron | B | BO3- B4O7- | 20 |
Chlorine | Cl | Cl | 100 |
Table 5-2: Essential macronutrient elements and form absorbed by most plants
Element | Symbol | Ions Most Frequently Absorbed | Conc. in Dry Tissue (ppm) |
---|---|---|---|
Nitrogen | N | NO3- NH4+ | 15000 |
Potassium | K | K+ | 10000 |
Calcium | Ca | Ca++ | 5000 |
Phosphorus | P | H2PO4- HPO4+ | 2000 |
Magnesium | Mg | Mg++ | 2000 |
Sulfur | S | SO4+ | 1000 |
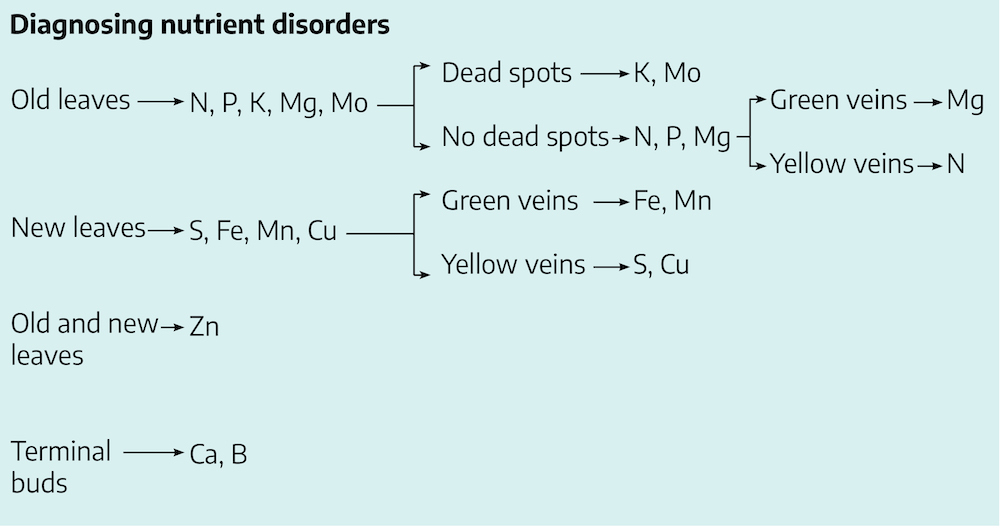
Functions of Nutrients and Diagnosing Deficiencies
Diagnosing nutrient deficiencies is not an easy task. Familiarity with a particular plant species helps since nutrient deficiencies are not always expressed the same in all plants. In addition, other environmental factors have to be considered because plants may express symptoms similar to nutrient deficiency when exposed to pathogens, insects, air pollutants, pesticides and abiotic stresses.
Chapter 6 “Diagnosing Plant Damage” outlines under “Key to Symptoms of Chemical Disorders,” the general descriptions of symptoms associated with nutrient deficiencies in plants.
Nutrient deficiencies are typically initiated in old leaves, new leaves or terminal buds. If terminal buds are dying or dead, and the leaves appear leathery, then the plant is likely deficient in either boron or calcium. If symptoms develop in both old and young leaves, then the element lacking is likely Zn. If symptoms show in the old leaves you can narrow the elements down to N, P, K, Mg or Mo. These elements are mobile in plant tissues. This means as the older leaves die, because of a lack of one of these elements, those elements that are remaining are moved out of those leaves to sustain the actively growing terminal meristems.
If symptoms develop in young terminal leaves then the deficiency is likely due to a lack of S, Fe, Mn, or Cu. These elements are considered to be immobile in plants since they are tightly bound to organic molecules in the healthy plant tissues and cannot be moved to the new leaves to support growth.
These groups of elements are further subdivided based on whether the leaves have dead spots and green or yellow veins.
Effects of pH on nutrient deficiencies and toxicities
pH is a measure of the hydrogen ion concentration in the soil (see the Soils Chapter for more details). The higher the hydrogen ion concentration the more acid is the soil. pH ranges from 0-14, with zero being the most acidic and 14 the most alkaline or basic. A pH of 7 is neutral and most plants grow best around 6.5 to 7. Some plants, like azaleas and rhododendrons, prefer a more acid pH and desert plants prefer a more alkaline pH.
Nutrient uptake by plants is affected by pH. Generally, an acid soil tends to make micronutrients more readily available to plants, and at extremes can result in toxic levels. The element Mo is the exception and its availability decreases. Macronutrients on the other hand are more available in slightly acid to slightly alkaline soils. “Figure 2-8: Nutrient availability by pH” in Chapter 2 demonstrates how pH affects nutrient availability. This chart does not represent all soil types since the chemistry, structure and composition of soils varies. Aberrations in uptake of some elements, such as B and P, is a result of the nature of the soil and complex chemical reactions that occur between soil particles and the element at different pH.
Physiological and biochemical functions of essential elements in plants
The list below reviews some of the functions elements have in plants. Limiting any single element would significantly limit plant growth and development. C, H, and O (not listed) make up the greatest percentage of elements in plants and represent the basic building blocks of plant tissues. Some elements are listed as beneficial since they have not been shown to be essential for growth in all plants. For example some plants may substitute Na for K to control osmotic relations in tissues. Others such as diatoms (a unicellular alga) have an absolute requirement for Si in the cell wall. Heavy metals are frequently toxic to plants, particularly under extremely acidic conditions.
Essential Elements
- Nitrogen (N): Constituent of many compounds, amino acids, nucleic acids, chlorophyll
- Phosphorus (P): Constituent of nucleic acids, phospholipids, ATP, NAD, NADP
- Potassium (K): Cofactor for enzyme reactions; osmotic balance particularly stomata
- Sulfur (S): Constituent of proteins; coenzyme A
- Calcium (Ca): Calcium pectate of middle lamella; important in membrane selectivity
- Magnesium (Mg): Constituent of chlorophyll, important in nucleic acid structure, coenzyme
- Iron (Fe): Constituent of cytochromes, ferredoxin; needed for chlorophyll synthesis
- Manganese (Mn): Coenzyme for numerous reactions; Krebs cycle, nitrate reductase
- Copper (Cu): Coenzyme – oxidases in plastocyanin a carrier in photosynthetic phosphorylation
- Zinc (Zn): Needed for IAA synthesis and protein synthesis; cofactor numerous dehydrogenases
- Molybdenum (Mo): Important in nitrate reduction and nitrogen fixation; probably also a cofactor
- Boron (B): Uncertain. Possibly involved in sugar translocation cross cell membranes, may alter hormone balance in plants
- Chlorine (Cl): Photosynthesis (deficiency never seen in nature)
Beneficial Elements
- Cobalt (Co) Nitrogen fixation
- Sodium (Na) Can partly replace K
- Selenium (Se) Can reverse P toxicity in susceptible plants
- Silicon (Si) Can improve growth in cereals. Reduces Fe, Mn toxicity by precipitation
Toxicity
- Most heavy metals are toxic.
- Low pH can lead to toxicity of Fe, Mn, Al particularly, in tropical soils.
Macronutrient deficiency outline
Nitrogen (N)
Phosphorus (P)
Potassium (K)
Magnesium (Mg)
Calcium (Ca)
Sulphur (S)
Micronutrient deficiency outline
The majority of the micronutrients are not mobile; thus, deficiency symptoms are usually found on new growth. Their availability in the soil is highly dependent upon the pH and the presence of other ions. The proper balance between the ions present is important, as many micronutrients are antagonistic to each other. This is especially true of the heavy metals where an excess of one element may show up as a deficiency of another. If the pH is maintained at the proper level and a fertilizer which contains micronutrients is used once a year, deficiency symptoms (with the exception of iron deficiency symptoms) are rarely found on indoor plants. Many of the micronutrients are enzyme activators.
Iron (Fe)
Boron (B)
Zinc (Zn)
Copper (Cu)
Manganese (Mn)
Molybdenum (Mn)
Chlorine (Cl)
Cobalt (Co)
Salinity Stress
The Origin of High Saline Soils
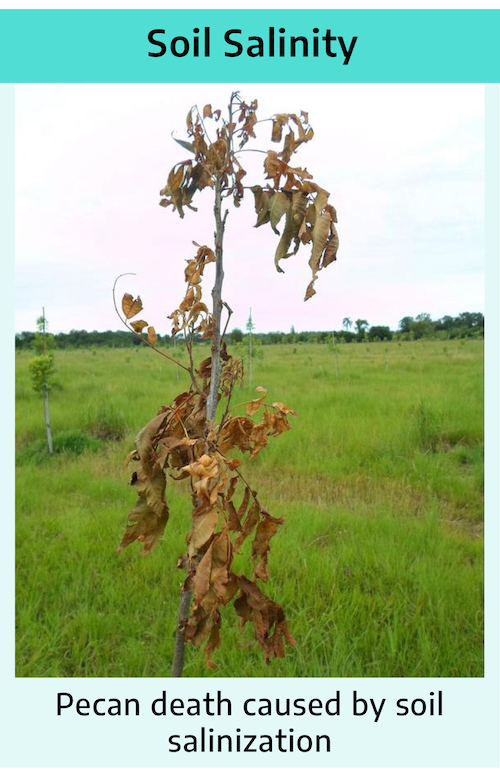
There are two types of salinity relative to how such soils are formed.
Primary salinity results from natural causes such as weathering and erosion or from land near the ocean that accumulates salt as a result of salt spray carried inland by wind and deposited in the soil by rain. Primary salinity can occur over very long periods of time depending on location and weather conditions.
Secondary salinity results from human activity such as irrigation and removal of natural vegetation and can occur much more quickly than primary salinity. Continuous irrigation and fertilization of agricultural areas results in a build up of salts in the top layers of the soil unless salts are leached out by rainfall or carefully controlled irrigation practices. The source of irrigation water may also have high levels of salts in the water that contribute to salt accumulation. Removal of natural vegetation often destroys deep-rooted perennials that have adapted to environments where water would be unavailable to shallow-rooted annual crop plants. Removing perennials and replacing them with annual crops often requires irrigation that ultimately raises the water table in the soil profile and exposes plants to higher salinities. Evaporation of high salinity irrigation water also contributes to increased soil salinity in the upper soil profile.
Differences Between Saline and Sodic Soils
Soils with high salt concentrations are further divided into saline and sodic soils. Saline soils have a high concentration of soluble salts, particularly sodium salts, whereas sodic soils have low concentrations of soluble salts. In sodic soils, monovalent cations such as sodium, displace divalent cations that hold clay particles together. This causes clay particles to dissociate and disperse. Over time dispersed particles are leached deeper into the soil profile-blocking pores in the soil leading to water logging and poor drainage. Alkaline soils are a type of sodic soil with a high pH and are typical of semi-arid to arid regions. Salts typical of saline soils include: NaCl, Na2SO4, while salts typical of sodic/alkaline soils are: Na2CO3, NaHCO3. Salts of the cations Na+, Ca2+, Mg2+, and K+, and the anions Cl–, SO42-, HCO3-, CO32-, and NO3- can also form in soils.
About 6.5% of the world’s soils are considered to be sodic or saline. Approximately 20% of all irrigated land is either sodic or saline.
Plant Responses to High Salinity Environments
Plants can be classified relative to salinity tolerance as glycophytes, halophytes, obligate halophytes, and facultative halophytes. Glycophtes cannot tolerate salinity levels in excess of 10mM (millimolar), halophytes can tolerate a concentration up to 50mM, obligate halophytes do not grow well below 10mM but grow well between 10-50mM, and facultative halophytes only become halophytic after being exposed to moderate soil salinity.
Two problems develop in plants as a result of high saline conditions. One is an osmotic problem due to high salt concentrations in the soil. This results in drought stress for the plant since water moves out of the plant into the soil. The other problem is one of toxic ion accumulation in the tissues of the plant.
The osmotic problem is countered in some plants by producing organic solutes in the cytoplasm that retain the water in the plant instead of allowing water to move into the soil. This requires metabolic expenditure of energy and thus slows the growth of the plant. Another adjustment the plant may make is the uptake of potassium ions or other low toxicity ions to counter the soil salt concentrations. Controlling ionic balance in the plant is critical in maintaining pH conducive to enzyme function and metabolism.
Toxic ion accumulation is prevented in some plants by exclusion by the roots, or prevention of transport in the shoots, to growing meristems of the plant. If salts reach the shoots and leaves, they can be compartmentalized in vacuoles or extruded by way of salt glands in some plants. If salts are not excluded or compartmentalized they can have deleterious effects on proteins and cell membranes and ultimately decrease growth and result in death. The following list summarizes some of the physiological effects of salinity stress on plants:
Salinity Stress Effects on Plants
- High Na+ transport to shoot
- Low K+ uptake
- High Cl– uptake
- Low P and Zn uptake
- Preferential accumulation of Na+ in old leaves
- Increase in non-toxic organic solutes
- Increase in free radical oxygen species
- Lower fresh and dry weight of shoots and roots
- Low germination of seeds
- Partial closure of stomata in response to water deficit
The tolerance of a plant depends on its ability to exclude, compartmentalize, or remove salt from tissues. In addition, adjusting the ion balance in the plant and the production of organic chemicals that help to retain water in the plant are fundamental to tolerance.
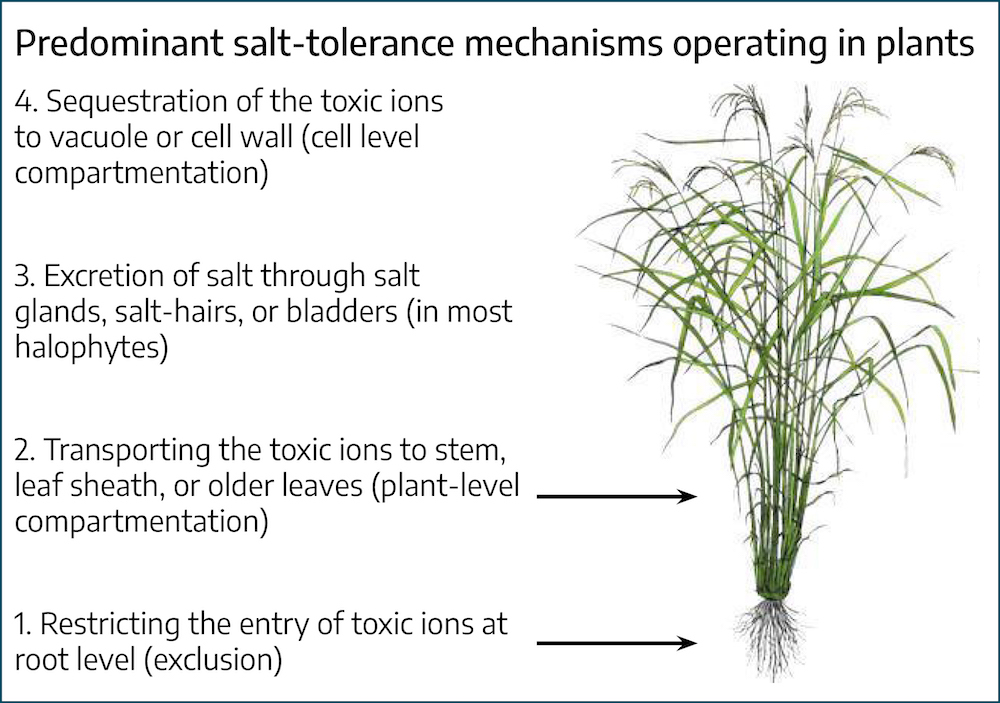
Symptoms of Salinity Stress in Plants
In the home landscape, like many other stresses, it is difficult to determine if the stress is caused by high salinity or some other stress presenting similar symptoms. One must carefully examine the environment in which the plant is growing to determine the surroundings of the plant in order to eliminate other possibilities.
Some of the symptoms of salinity stress in plants include: reduced shoot growth, marginal necrosis of leaves, delay in bud break and flowering, premature defoliation, early leaf color formation, crown thinning, and twig death.
Salt injury to plants along roadways is common as a result of using salts to melt snow and ice. Sources of salt damage can include salt spray from passing vehicles or salt that has seeped into the soil around plant roots. Symptoms usually start to show in the early to late spring, depending on the amount of salt to which the plant is exposed, soil types and weather conditions. Symptoms may not develop for quite some time if soils are affected. Homeowners using salt on driveways and sidewalks may also experience salt damage to their plants. Watering plants with softened water may also contribute to salt injury in sensitive plants.
Herbicide Injury
Herbicide injury to landscape plants is most likely a result of using herbicides on lawns unless you live near an agricultural production area.
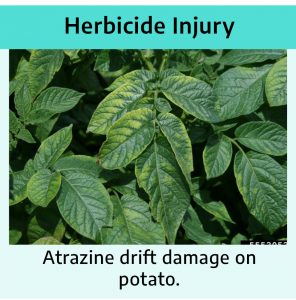
There are several ways non-target plants can be exposed to herbicides and include:
- Drift and volatilization: Herbicides should never be applied on windy days because they can drift to non-target plants and cause injury. Some pesticides are volatile and can vaporize in the hot sun. The vapors are then carried by wind to other plants. When applying herbicides, spray particle size is also important. The smaller the particle size of the spray, the more likely it can be carried by the wind or be vaporized.
- Leaching and run-off from the soil: Some herbicides are applied to soils and are referred to as pre-emergence herbicides. These form a thin layer of chemical on the soil and when newly germinating seedlings attempt to break through the soil, they are killed by the herbicide. Some of these chemicals can run off in rain water and affect other plants or potentially leach down through the soil and possibly be taken up by deep-rooted plants such as trees.
- Misapplication: Bottom line, read the label on the product you are using. Only use the herbicide on plants listed on the label and follow the recommended rates.
- Soil or soil amendment application: Make sure you know the history and source of any top soils or soil amendments you may use around plants. Be particularly aware of agricultural fertilizers and compost that may have originated from previous treatment of hay fields with weed control herbicides. These can be carried over in livestock manure and compost.
Herbicide Application and Effects on Plants
Herbicides are either applied to soils (pre-emergence) to control newly germinating seedlings or directly to shoots and foliage (post-emergence) to control already established growing plants. Herbicides can further be divided based on whether the chemical affects the plant at the site of application (contact) or whether it moves around in the vascular system of the plant (systemic). Herbicides can be selective, that is some will kill only broadleaf plants or only grasses, or they can be non-selective and kill both. Factors such as how the herbicide is used (pre/post-emergence), and whether it is a contact or systemic herbicide, or whether it is selective or non-selective has to do with the herbicide’s mode of action. The mode of action refers to how a chemical affects the physiology, biochemistry, and development of the plant.
Differentiating herbicide damage in plants from other environmental stresses is sometimes difficult. Nutrient, air pollution, salinity, pathogens, insects and other environmental stress can sometimes mimic herbicide damage symptoms. However, if one can eliminate these other potential stress factors, knowledge of herbicide modes of action can be helpful in determining if a specific herbicide may have caused the damage.
Herbicide Modes of Action
The following outlines the different categories of herbicides relative to their mode of action and how they affect plant metabolism.
- Amino acid synthesis inhibitors prevent the synthesis of aromatic and branched chain amino acids required for the synthesis of enzymes and membrane proteins.
- Plant growth regulator herbicides mimic or disrupt auxin (indole acetic acid) functions in plants. Auxin controls many aspects of plant development including: cell division, sex, tropisms, and polarity.
- Pigment inhibitors prevent the formation of carotenoids in plants that are crucial for protection of chlorophyll from photodecomposition. These are also called bleaching herbicides because the leaves of treated plants are white.
- Photosynthesis inhibitors prevent the light reactions of photosynthesis from functioning. Photosynthesis is divided into two phases: the light reactions, which captures the energy of light (photons) and stores it as stable chemical energy, and the dark reactions, which uses the energy produced in the light reactions to make sugar.
- Cell membrane disruption herbicides oxidize membranes directly and cause membranes to break down and decompose. Contact herbicides are examples.
- Seedling growth inhibitors affect apical cell division of roots and shoots.
- Lipid synthesis inhibitors affect plant cell membranes and membranes surrounding chloroplasts, mitochondria, vacuoles and other organelles in the cell. This disrupts the ability of each to perform a specific function.
- Nitrogen metabolism inhibitors affect the metabolism of ammonium into amino acids, which can accumulate to toxic levels in the plant.
The VCE Pest Management Guide: Home Grounds and Animals lists a variety of herbicides that are approved for use on Virginia lawns. Most of these are used to control broadleaf weeds, nuisance grasses, and sedges. Although there is a possibility that any of these could be used in lawns, it is most probable that the plant growth regulator (PGR) type herbicides are going to be the most prominent since they are found most frequently in weed and feed fertilizers. So where weed and feed fertilizers are used in lawn care and non-target plants show injury symptoms, the most likely suspect would be the PGR group of herbicides.
Physical Stress
The Importance of Water to Plants
Water is important to plants for maintaining tissue turgidity. That means there needs to be an internal water content that allows cells and tissues to maintain maximum cell volume and thus tissue surface area. A tissue that is not turgid is said to be flaccid and often is accompanied by wilting. Wilting is a defensive mechanism to reduce water loss from the plant, but it also reduces leaf surface area to sunlight and thus reduces photosynthesis and growth (for more information on this topic, see the Chapter 1 “Botany”).
Water is also important for transporting nutrients from the soil to the tissues of the plant. The xylem is the main water and nutrient transport system, extending from the roots through the stems and into the veins of the leaf. In the leaf, water is lost through the stomata when they are open during the day, thus providing another important function for water and that is cooling the leaf surface through the process referred to as evapo-transpiration. Water is also required for photosynthesis.
Drought Stress
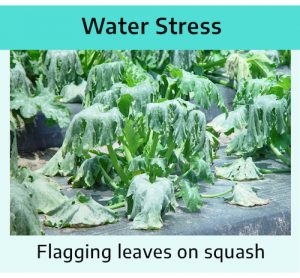
Causes of drought stress in plants
High temperature, low soil moisture, low humidity, wind, frozen soil, high salt concentrations and low genetic tolerance to moisture stress all contribute to water deficit stress in plants. Many of these stresses can act at the same time and frequently do. Each deprives the plant of moisture through the lack of availability as in low soil moisture and frozen soils, or through moisture removal from the plant as a result of high salt concentrations, high wind, and low humidity.
Plant control of water loss: Physiological/developmental responses
One of the primary ways plants control water loss is through opening and closing stomata on the surface of leaves. Stomata in most plants are open during the day and close at night. The exception being: plants growing in hot dry climates such as CAM plants that open stomata at night to conserve precious water during the day. However, most plants under water deficit stress can partially or totally close stomata during the day to conserve water. Other responses plants have include leaf drop, flower drop, and fruit drop, early flowering, die back in trees, increased root growth, wilting/leaf rolling and internal production of molecules in cells that help retain water. All of these reflect survival strategies at the expense of growth.
Plant control of water loss: Genetic adaptations for conserving water
Plant adaptations to low moisture environments include: More stomata on under surface of leaves than on the top, sunken stomata, thick leaf cuticle and high wax production, modified leaves (no leaves to narrow pointed leaves), hairy and light-colored leaf surfaces, low plant profile, different strategies for opening and closing stomata, and leaf movements to avoid direct sun exposure.
Water use in plants
Plants are part of the hydrologic cycle and are important in recycling of water from the soil to the air. Some plants are more efficient at water usage than others. It has been estimated that about 1/3 of the rainfall that falls on a forested ecosystem is transpired through evapotranspiration back to the atmosphere. Generally, C4 plants are more efficient than C3 plants in water use. However, even a C4 plant, such as corn, uses considerable amounts of water to grow. It has been estimated that it takes about 16.6 gallons of water to produce an ear of corn, and at a planting rate of about 30,000 plants per acre, that translates to 500,000 gallons per acre not including the water needed for the vegetative growth of the corn stalk.
Trees also transpire large amounts of water during the summer. For example, a 46’ silver maple tree can transpire as much as 60 gallons per hour.
Flooding Stress
Effects of flooding on soils
When a soil is saturated with water after a heavy rain or during a flood, it can impact plant growth severely. Short periods of flooding can have significant impacts on crops and plants in general. Flooding deprives plants of O2 (anaerobic conditions) in the root system and prevents CO2, due to respiration, from escaping from the soil into the air. This creates an O2 deficit in the soil and inhibits root growth. As CO2 builds up, carbonic acid is formed from the reaction of CO2 with water making the soil more acidic. In so doing, it makes some elements in the soil more readily available sometimes in toxic amounts. Other essential elements such as nitrogen can be leached from the soil or made less available because of acidic conditions. In addition, anaerobic bacteria become more active, producing toxic metabolites such as methane, ethylene, and hydrogen sulfide. These conditions weaken the plant root system, making it more susceptible to root pathogens. This scenario can also be applied to overwatering of potted plants in the home or nursery.
Physiological changes in flooded plants
Flooding puts plants into a survival mode rather than a growth mode. Plants respond by growing adventitious roots on the stem near the water line thus being closer to available atmospheric O2. Ethylene production increases, which is a natural plant hormone that inhibits growth rate and induces a condition called epinasty in the leaves. Plants showing epinasty appear wilted. This is not wilting as a result of lack of available water but rather a loss of turgidity of petiole basal cells in response to ethylene. In woody plants, flooding causes an increased production of openings in the bark called lenticels, which facilitate gaseous exchange. Also, plants that are flooded for extended periods produce a type of tissue in the roots called aerenchyma, which is a degradation of root cellular structure in order to facilitate movement of O2 among plant tissues and removal of CO2 . Interestingly, plants that normally live in aquatic environments, such as cattails, normally have this type of tissue in root systems and stems. Finally, some plants that normally live in aquatic environments such as mangroves, produce structures called pneumatophores, which have been shown to be involved with O2 transfer to the roots of these plants. Analogous structures in cypress do not appear to have that function.
Heat Stress
Water deficit stress is most frequently linked to high temperature or heat stress. All plants have an optimum temperature for good growth. Exceeding that temperature can have profound effects on the physiology and biochemistry of the plant. When temperature exceeds the optimum for a given plant, a number of things start to happen. Stomata start to close or shut down completely to limit water loss. However, this limits CO2 uptake, causing a reduction in photosynthesis. Also, O2 cannot get out of the leaves and builds up in the leaf tissues and can result in the formation of free radical O2, which oxidizes cell membrane lipids, making them leaky and non-functional. Respiration increases in the cells, causing an increased use of stored energy in the plant thus resulting in reduced growth and possibly death of the plant depending on the severity and length of exposure.
Plants tolerant to heat stress are capable of altering their membranes to protect from oxidation. They also produce molecules (heat shock proteins) that can be incorporated into plant membranes or used to help conserve cellular water and make them less susceptible to heat stress.
Chilling and Freezing Stress
The Difference Between Chilling and Freezing Stress
Chilling stress occurs in plants sensitive to temperatures in the range of 68-32° F. These include plants that are tropical to subtropical in origin, such as many indoor species, beans, corn, rice, tomatoes, squash and bananas.
Freezing stress occurs in plants sensitive to temperatures below the freezing point of water or 32°F. Some plants are frost hardy and can acclimate to temperatures just below freezing and can recover if the temperature is not too prolonged. Peas, spinach, lettuce are examples of such plants.
Other plants are cold hardy and include temperate trees and woody shrubs, some of which can with withstand temperatures of -70°F and lower.
Physiological and Biochemical Differences Between Cold Sensitive and Tolerant Plants
Several things are involved biochemically and physiologically that separate plants that are sensitive from those that are tolerant to cold temperatures. Plants that are freezing tolerant can prevent ice from forming inside the cell. If this occurs in plants, it is lethal. Plants that are susceptible to freezing temperatures cannot do this. Cold tolerance is accomplished through changes in membrane composition and the production of substances inside the cell that lower the freezing point.
Structurally the plant cell is comprised of an outer cell wall comprised of cellulose, and immediately inside the cell wall is the cytoplasmic membrane. The membrane controls the movement of water and dissolved substances in and out of the cell. Membranes also surround important organelles inside the cell, including chloroplasts, mitochondria, vacuoles as well as many other structures. Plant membrane composition changes with temperature. Membranes are comprised primarily of lipid (with some protein and carbohydrate), and lipids can change viscosity with changing temperature. That is, they become more fluid-like with elevated temperature and more solid-like at lower temperatures. The challenge for the plant is to control how fluid or how solid the membrane becomes, because this affects the ability of the membrane to transport water and dissolved substances across it. Plants can control this fluid/solid nature by interjecting unsaturated or saturated fats into the membrane structure. If you have ever baked pastries you have experienced saturated and unsaturated fats. An example of an unsaturated fat is vegetable oil, which is liquid at room temperature. On the other hand, lard is a saturated fat and is solid at room temperature. So this is basically what you find in plant membranes: combinations of saturated and unsaturated fats that change with temperature to affect membrane permeability and function when it gets too hot or too cold. So when plants experience increasing cold temperatures, they interject unsaturated fatty acids so as to maintain a more fluid membrane, which is functional at lower temperatures. Conversely, when it gets hot, membranes can become more permeable and the plant injects more saturated fats into the membrane to make it less fluid. This is basically what happens when you harden off your tomato plants in early spring. The ability to make such changes in membrane structure is genetically determined and is part of the basis for differences in temperature sensitivity in plants.
Along with membrane changes, plant cells also produce substances called sugar alcohols that lower the freezing point of the cytoplasm so it does not form ice crystals. Some plants have the ability to move water out of the cell into the area of the cell wall where freezing is less likely to damage the cell. When ice starts to form, however, water diffuses out of the cell to form more ice. This may be both beneficial or harmful depending on how much water is removed. Some plants, mostly trees, undergo a process called supercooling where water can stay liquid down to a temperature of -70°F.
The Symptoms, Cellular, and Physiological Effects of Chilling and Freezing
Symptoms: reduced growth, leaf necrosis or damage, water soaking and a flaccid appearance of plant tissues.
Cellular effects: loss of membrane function, dehydration, salt injury, membrane rupture as a result of ice formation, air bubbles(embolisms) in the xylem.
Physiological effects: decreased photosynthesis, protein degradation, reduced transport in vascular tissues, increased respiration.
Light Stress
Light is important in promoting photosynthesis, affecting plant orientation, promoting/inhibiting flowering, germination and dormancy, stomatal opening and closing and developmental processes (photomorphogenesis).
Plants can either be exposed to too much light, too little light (quantity), or the wrong kind of light (quality). Some plants are considered to be sun plants in that they grow well in full sunlight while others prefer shade or partial sun. Characteristics of sun plants include: reduced leaf area, thick leaves and cuticle, and abundant stomata. Shade plants have the opposite characteristics.
The quality of light is important as well in that red and blue wavelengths of light are needed for photosynthesis, phototropism (growth of plants toward light), stomatal opening, and solar tracking (plants adjusting leaves during the day to follow the sun). Red light and far-red light are important in flowering, seed germination and dormancy. UVB light can damage plants and inhibit growth. This is particularly true at higher elevations.
Plants growing in the under-story of a forest have the ability to capture what little light that may penetrate the canopy in a very efficient way. When walking through the forest on a sunny, slightly windy day, you may have noticed the flickers of light that come and go with the tree canopy movements. These flickers of light are referred to as sun flecks and are important in the growth of under-story plants.
Mechanical Stress
Mechanical stress includes wind, rain, hail, snow, ice and animal movement. Plants can sense touch or movement that may be caused by any of the above mechanical stimuli. In some cases the response is not obvious, but in others such as the Venus fly trap (Dionaea muscipula) or the sensitive plant (Mimosa pudica), perception of touch can be very rapid and obvious.
Effects of Mechanical Stress on Plants
Wind is probably one of the most prevalent mechanical stresses and causes plants to respond in several ways. Wind can be beneficial as well as harmful to plants. When wind is strong it can cause physical damage to the plant but subtle and less obvious effects are changes in photosynthesis. On a calm clear warm day, plants have their stomata open and O2 from photosynthesis diffuses out and CO2 goes in. However, on calm days a layer of high humidity produced from evaporative transpiration forms near the surface of leaves called a boundary layer. This layer creates a resistance to the exchange of gases between the atmosphere and the leaf, slows down photosynthesis, and retains O2, which slows growth. Consequently a slight breeze can be beneficial to plants in that the boundary layer is removed and allows gas exchange more efficiently.
However, if the wind is rapid it could create a water deficit stress for the plant. The stomata close and again photosynthesis is reduced and growth is slowed.
There is still another effect that is a result of constant flexing and movement of plant leaves and branches as a result of wind or other mechanical stimuli. This results in chemical signals that are transmitted in the tissues of the plant causing changes in growth patterns, usually reducing the height of plants and increasing the diameter of shoots or branches. If you have ever observed trees growing on mountaintops, you have observed the effects of wind on growth. Such plants are usually small and misshapen, reflecting the wind direction.
Practical applications of this knowledge have been used in fruit and nursery production. For example, attaching weights to fruit tree branches or bending branches down and tying them to the ground causes the branches to increase in diameter and shortens elongation growth, thus providing a stronger branch that is capable of supporting a larger, heavier fruit load without causing damage to the tree. Researchers have also shown that by using mechanical devices, which gently pass across the tops of greenhouse-grown plants, they can cause decreases in height growth and increased stem diameter. This is beneficial to growers in that it prevents plants from becoming leggy and better able to support inflorescences. It also avoids the use of plant growth regulator chemicals often used for this purpose.
Rain, hail, snow and ice can cause observable mechanical damage to plants, such as leaf tearing/removal and limb damage but can also induce similar responses as wind, causing changes in growth distribution in tissues. However, these conditions are usually short term as opposed to almost daily plant encounters with wind.
Effects of Soil Compaction on Root and Seedling Growth
Soil compaction also impacts differential growth in plant tissues, particularly the roots and germinating seeds. Persistent pedestrian traffic, animal grazing, construction machinery and the nature of the soil can cause soil compaction. Compaction can affect many things that influence plant growth including gas exchange in the soil and water and nutrient movement. However, soil compaction also imparts a physical resistance to the growth of plant roots and the growth and penetration of germinating seedlings. This resistance causes roots and shoots to increase in diameter in a similar fashion to wind stress. This is thought to give the root or young shoot a greater mechanical advantage to penetrate and grow through a compacted soil. Certainly soil compaction will affect growth and survival of plants depending on plant species.
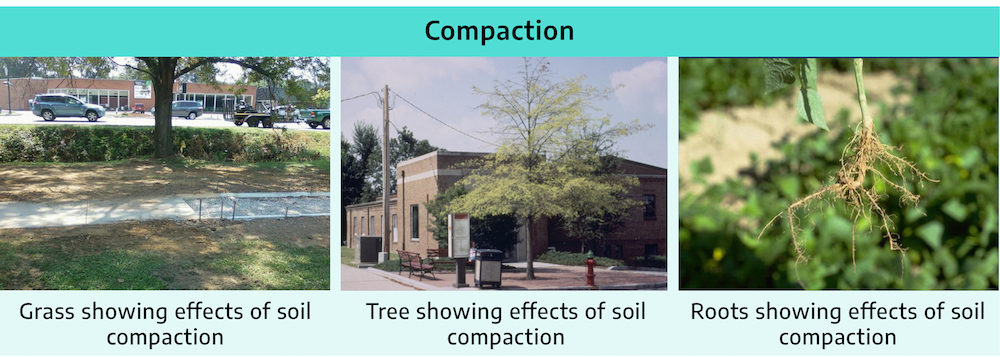
Summary of Physical Stresses
We now know that creating and defining microclimates in crops (wind breaks/terraces) and in urban landscapes (plantings around buildings etc.) can influence the physiology of plants in favorable and unfavorable ways. So when considering all of the physical and chemical stresses plants must endure to grow well, it is important that we know as much about how these stresses impact plants as we can, so we can make the best decisions for optimum plant growth.
Additional Resources
Acid Rain:
- Effects of Acid Rain, U.S. EPA
- National Air Quality: Status and Trends of Key Air Pollutants, U.S. EPA
Nutrient deficiency:
Salinity:
Herbicide damage:
Light stress:
Physical stress:
References:
Reich, P.B. et al. (2018). Unexpected reversal of C3 versus C4 grass response to elevated CO2 during a 20-year field experiment. Science 360(6386), 317-320.
Attributions
Written by David M. Orcutt, Professor Emeritus, Plant Physiology (2015)
- Reviewed by Daivid M. Orcutt, Professor Emeritus, Plant Physiology (2021)
Image Attributions
- Figure 5-1: Factors affecting plant growth and development. Johnson, Devon. 2022. CC BY-NC-SA 4.0 Adapted from “Figure 18.1” in “Signal Perception and Transduction” by A. Trewavas. Biology. 2002.
- Figure 5-2: Environmental stressors effects’ on plants, response, and result. Johnson, Devon. 2022. CC BY-NC-SA 4.0. Adapted from “Risposta delle colture in serra agli stress ambientali” by Anonymous, 2018.
- Figure 5-3: Carbon dioxide over 800,000 years. NOAA Climate.gov image from “Climate Change: Atmospheric Carbon Dioxide” 2022. Public domain.
- Figure 5-4: Atmospheric carbon dioxide (1960-2021). NOAA Climate.gov image from “Climate Change: Atmospheric Carbon Dioxide” 2022. Public domain.
- Figure 5-5: Sulfur dioxide injury on sumac. Johnson, Devon. 2022. CC BY-NC-SA 4.0 Includes image 1634216 by Penn State Department of Plant Pathology & Environmental Microbiology Archives from Bugwood.org CC BY-NC 3.0 US.
- Figure 5-6: Diagnosing nutrient disorders. Johnson, Devon. 2022. CC BY-NC-SA 4.0. Adapted from Master Gardener Training Handbook, Virginia Cooperative Extension, 2018.
- Figure 5-7: Pecan death caused by soil salinization. Johnson, Devon. 2022. CC BY-NC-SA 4.0 Includes image 5545229 by Jonas Janner Hamann from Bugwood.org CC BY 3.0 US.
- Figure 5-8: Predominant salt-tolerance mechanisms operating in plants. Johnson, Devon. 2022. CC BY-NC-SA 4.0. Adapted from “Predominant salt tolerance mechanisms operating in plants,” Knowledge Bank.
- Figure 5-9: Atrazine drift damage on potato. Johnson, Devon. 2022. CC BY-NC-SA 4.0. Includes image 5553953 by Jed Colquhoun from Bugwood.org CC BY-NC 3.0 US.
- Figure 5-10: Flagging leaves on squash plants indicate water stress. Johnson, Devon. 2022. CC BY-NC-SA 4.0 Includes image 5602899 by Gerald Holmes from Bugwood.org CC BY-NC 3.0 US.
- Figure 5-11: Soil compaction and root damage, tree showing signs of abiotic stress from soil compaction in urban area, dry bean roots showing the effects of soil compaction. Johnson, Devon. 2022. CC BY-NC-SA 4.0. Includes image 5334099 by Mary Ann Hansen from Bugwood.org CC BY 3.0 US, image 5454888 by Jason Sharman from Bugwood.org CC BY-NC 3.0 US, and image 5358725 by J.G. Davis from Bugwood.org CC BY 3.0 US.
Any change in environmental conditions that adversely affects survival, growth, development and yield in plants
Found in temperate to cold climates with high moisture environments and represent the majority of plant species. They typically are slower-growing plants than C4 plants and use CO2 less efficiently as a result of an energy-wasting process called photorespiration.
Found in hot, dry, subtropical to tropical environments. They are fast growers and have higher rates of photosynthesis than C3 plants. Because C4 plants do not photorespire, they are more efficient in fixing CO2 than C3 plants.
Found in very hot dry desert areas. Like C4 plants, CAM plants are not susceptible to photorespiration because, unlike C3 and C4 plants, they open their stomata at night, thus conserving water and fixing CO2 and storing it for use during the day to make sugar.
Water lost through stomata when they are open during the day
Stress that occurs in plants sensitive to temperatures in the range of 68-32° F
Stress that occurs in plants sensitive to temperatures below the freezing point of water or 32°F