16 Plasma Membrane
Learning Objectives
- Describe the structure and functions of cellular membranes.
- Describe the chemical components of cell membranes and review their chemical properties.
- Explain carbohydrate involvement in membrane structure, and their possible functions and location.
- Describe the types of proteins found in membranes and the roles they play in membrane function.
- Stress the importance of membrane fluidity to living cells and the mechanisms by which cells maintain an appropriate level of fluidity.
- Describe biological membrane asymmetry and the dynamic nature of membrane structure and function.
- Describe the different mechanisms employed by cells to transport materials across membranes: simple and facilitated diffusion, channel proteins, and active transport.
About this Chapter
Plasma membranes must allow certain substances to enter and leave a cell, and prevent some harmful materials from entering and some essential materials from leaving. In other words, plasma membranes are selectively permeable — they allow some substances to pass through, but not others. If they were to lose this selectivity, the cell would no longer be able to sustain itself, and it would be destroyed. There are four major types of transport across the cell membrane:
- Diffusion,
- Diffusion through a channel,
- Facilitated diffusion (selective binding), and
- Active transport (requires ATP).
16.1 Components and Structure
A cellʼs plasma membrane defines the cell, outlines its borders, and determines the nature of its interaction with its environment. Cells exclude some substances, take in others, and excrete still others, all in controlled quantities. The plasma membrane has many significant functions:
- It must be very flexible to allow certain cells, such as red and white blood cells, to change shape as they pass through narrow capillaries.
- It carries markers that allow cells to recognize one another, which is vital for tissue and organ formation during early development, and which later plays a role in the immune responseʼs “self” versus “nonself” distinction.
- It functions as a medium for complex, integral proteins, and receptors to transmit signals. These proteins act both as extracellular input receivers and as intracellular processing activators.
- It imparts selective permeability essential for maintaining a resting membrane potential and osmoregulation.
Fluid mosaic model
The fluid mosaic model describes the plasma membrane structure as a mosaic of components — including phospholipids, cholesterol, proteins, and carbohydrates — that gives the membrane a fluid character. Plasma membranes range from 5 to 10 nm in thickness (figure 16.1). The protein, lipid, and carbohydrate proportions in the plasma membrane vary with cell type.
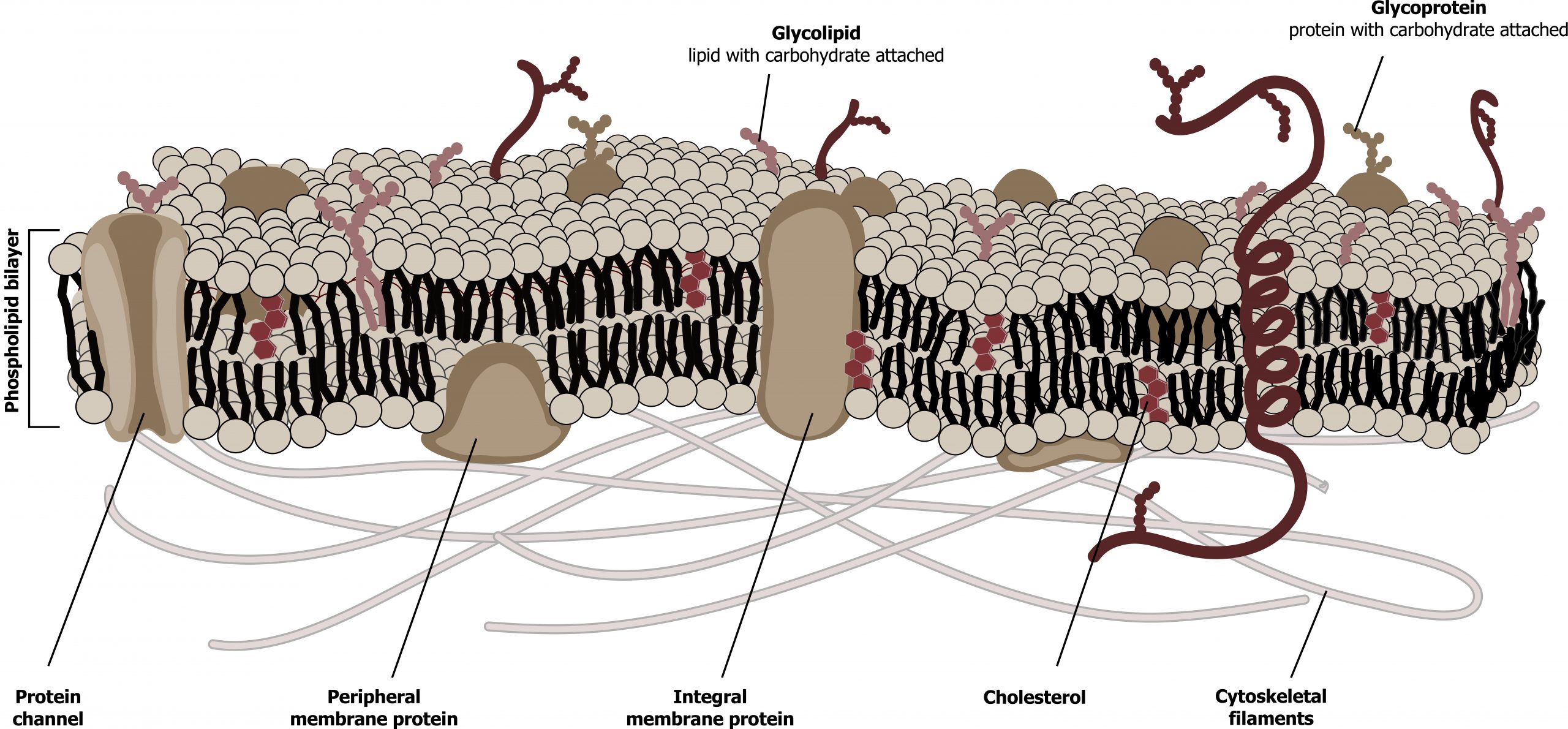
Lipids
The membraneʼs main fabric comprises amphiphilic, phospholipid molecules. The hydrophilic or “water-loving” areas of these molecules (figure 16.2) are in contact with the aqueous fluid both inside and outside the cell. Hydrophobic or “water-hating” molecules tend to be nonpolar. They interact with other nonpolar molecules in chemical reactions, but generally do not interact with polar molecules. A phospholipid molecule consists of a three-carbon glycerol backbone with two fatty acid molecules attached. This arrangement gives the overall molecule a head area (the phosphatecontaining group), which has a polar character or negative charge, and a tail area (the fatty acids), which has no charge. The head can form hydrogen bonds, but the tail cannot (figure 16.2).
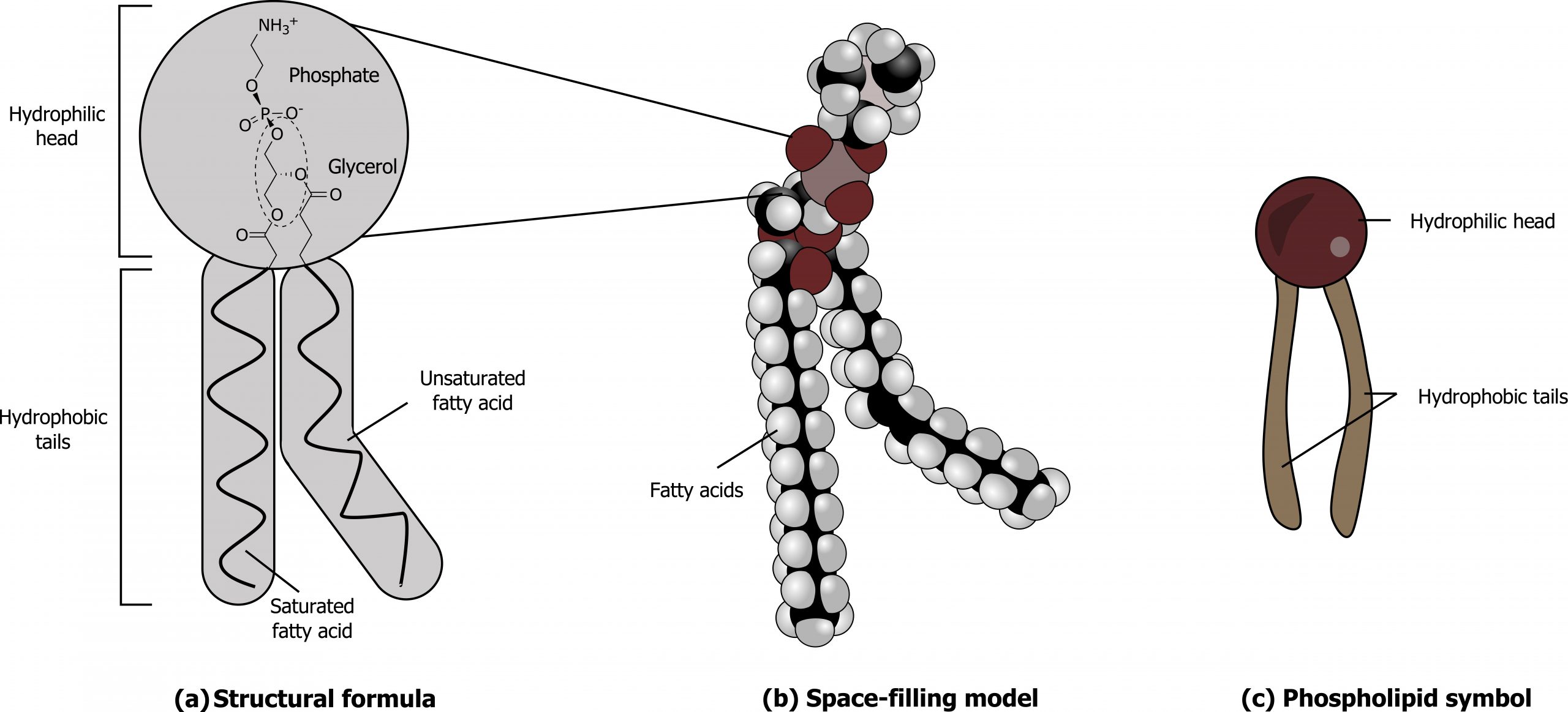
Cholesterol, another lipid comprised of four fused carbon rings, is situated alongside the phospholipids in the membraneʼs core (figure 16.2).
Specific phospholipids play key roles in the membrane; phosphatidylcholine, serine, inositol, and ethanolamine (figure 16.3) play various roles in the membrane.
![(a) Phosphoglycerolipids; Phosphatidic acid (PA) IUPAC ID: [(2R)-2-octadecanoyloxy-3-phosphonooxypropyl] octadecanoate. Phosphatidylserine (PS) IUPAC ID: (2S)-2-amino-3-[[(2R)-2,3-di(octadecanoyloxy)propoxy]-hydroxyphosphoryl]oxypropanoic acid. Phosphatidylethanolamine (PE). Phosphatidylcholine (PC). Phosphatidylinositol (PI). (b) Sphingolipids; Ceramide IUPAC ID: N-[(E,2S,3R)-1,3-dihydroxyoctadec-4-en-2-yl]butanamide. Sphingomyelin IUPAC ID: [(E,2S,3R)-2-(docosanoylamino)-3-hydroxyoctadec-4-enyl] 2-(trimethylazaniumyl)ethyl phosphate and is a phosphosphingolipid. Cerebroside IUPAC ID: (2R)-2-hydroxy-N-[(2S,3R,4E,8E)-3-hydroxy-9-methyl-1-[(2R,3R,4S,5S,6R)-3,4,5-trihydroxy-6-(hydroxymethyl)oxan-2-yl]oxypentadeca-4,8-dien-2-yl]octadecanamide and is a glycolipid.](https://pressbooks.lib.vt.edu/app/uploads/sites/66/2021/11/16.3-1024x472.png)
- Phoshatidylcholine is primarily found on the outer leaflet, while phosphatidylserine, inositol, and ethanolamine are all located predominately on the inner leaflet.
- Both phosphatidylserine and inositol are negatively charged at physiological pH allowing for binding of positively charged molecules.
- Sphingolipids and glycolipids are less abundant and play key roles in nervous tissue membranes. Disorders of sphingolipid or glycolipid metabolism are often severe (e.g., Tay-Sachs or Neimann-Pick disease).
Proteins
Proteins comprise the plasma membrane’s second major component. Integral proteins, or integrins, as their name suggests, integrate completely into the membrane structure, and their hydrophobic membranespanning regions interact with the phospholipid bilayerʼs hydrophobic region.
Peripheral proteins are on the membrane’s exterior and interior surfaces, attached either to integral proteins or to phospholipids. Peripheral proteins, along with integral proteins, may serve as enzymes, as structural attachments for the cytoskeletonʼs fibers, or as part of the cellʼs recognition sites. Scientists sometimes refer to these as “cell-specific” proteins. The body recognizes its own proteins and attacks foreign proteins associated with invasive pathogens. Additional proteins can be lipid anchored on the exterior of the membrane.
Carbohydrates
Carbohydrates are the third major plasma membrane component. They are always on the cell’s exterior surface and are bound either to proteins (forming glycoproteins) or to lipids (forming glycolipids). These carbohydrate chains may consist of two to sixty monosaccharide units and can be either straight or branched. Along with peripheral proteins, carbohydrates form specialized sites on the cell surface that allow cells to recognize each other. We collectively refer to these carbohydrates on the cellʼs exterior surface — the carbohydrate components of both glycoproteins and glycolipids — as the glycocalyx (meaning “sugar coating”). The glycocalyx is highly hydrophilic and attracts large amounts of water to the cellʼs surface. This aids in the cellʼs interaction with its watery environment and in the cellʼs ability to obtain substances dissolved in the water.
Membrane fluidity
The integral proteins and lipids exist in the membrane as separate but loosely attached molecules. The membraneʼs mosaic characteristics explain some but not all of its fluidity. There are two other factors that help maintain this fluid characteristic.
One factor is the nature of the phospholipids themselves. In their saturated form, the fatty acids in phospholipid tails are saturated with bound hydrogen atoms. There are no double bonds between adjacent carbon atoms. This results in tails that are relatively straight. In contrast, unsaturated fatty acids do not contain a maximal number of hydrogen atoms, but they do contain some double bonds between adjacent carbon atoms.
Temperature can also influence membrane rigidity. Decreasing temperatures compress saturated fatty acids with their straight tails, and they press in on each other, making a dense and fairly rigid membrane. If unsaturated fatty acids are compressed, the “kinks” in their tails elbow adjacent phospholipid molecules away, maintaining some space between the phospholipid molecules. This “elbow room” helps maintain fluidity in the membrane at temperatures at which membranes with saturated fatty acid tails in their phospholipids would “freeze” or solidify.
16.1 References and resources
Text
Clark, M. A. Biology, 2nd ed. Houston, TX: OpenStax College, Rice University, 2018, Chapter 4: Cell Structure, Chapter 5: Structure and Function of the Plasma Membranes.
Karp, G., and J. G. Patton. Cell and Molecular Biology: Concepts and Experiments, 7th ed. Hoboken, NJ: John Wiley, 2013, Chapter 4: The Structure and Function of the Plasma Membrane.
Le, T., and V. Bhushan. First Aid for the USMLE Step 1, 29th ed. New York: McGraw Hill Education, 2018, 49.
Figures
Grey, Kindred, Figure 16.1 Schematic of the cell membrane. Plasma membranes range from 5 to 10 nm in thickness. 2021. https://archive.org/details/16.1_20210926. CC BY 4.0. Added Cell membrane detailed diagram blank by LadyofHats. Public domain. From Wikimedia Commons.
Grey, Kindred, Figure 16.2 Structure of a phospholipid. 2021. Chemical structure by Henry Jakubowski. https://archive.org/details/16.2_20210926. CC BY-SA 4.0. Added Cell membrane detailed diagram 4 vi by P.T.Đ. CC BY-SA 4.0. From Wikimedia Commons.
Grey, Kindred, Figure 16.3 Important membrane lipids. 2021. Chemical structure by Henry Jakubowski. https://archive.org/details/16.3_20210926. CC BY 4.0.
16.2 Passive Transport
Plasma membranes must allow certain substances to enter and leave a cell, and prevent some harmful materials from entering and some essential materials from leaving. In other words, plasma membranes are selectively permeable; they allow some substances to pass through, but not others. If they were to lose this selectivity, the cell would no longer be able to sustain itself, and it would be destroyed. There are four major types of transport across the cell membrane:
- Diffusion,
- Diffusion through a channel,
- Facilitated diffusion (selective binding), and
- Active transport (requires ATP).
Recall that plasma membranes are amphiphilic: they have hydrophilic and hydrophobic regions. This characteristic helps move some materials through the membrane and hinders the movement of others.
Nonpolar and lipid-soluble material with a low molecular weight can easily slip through the membraneʼs hydrophobic lipid core. Substances such as the fat-soluble vitamins A, D, E, and K readily pass through the plasma membranes in the digestive tract and other tissues. Fat-soluble drugs and hormones also gain easy entry into cells and readily transport themselves into the bodyʼs tissues and organs. Oxygen and carbon dioxide molecules have no charge and pass through membranes by simple diffusion.
Polar substances present problems for the membrane. While some polar molecules connect easily with the cellʼs outside, they cannot readily pass through the plasma membraneʼs lipid core.
Additionally, while small ions could easily slip through the spaces in the membraneʼs mosaic, their charge prevents them from doing so. Ions such as sodium, potassium, calcium, and chloride must have special means of penetrating plasma membranes. Simple sugars and amino acids also need the help of various transmembrane proteins (channels) to transport themselves across plasma membranes.
Diffusion
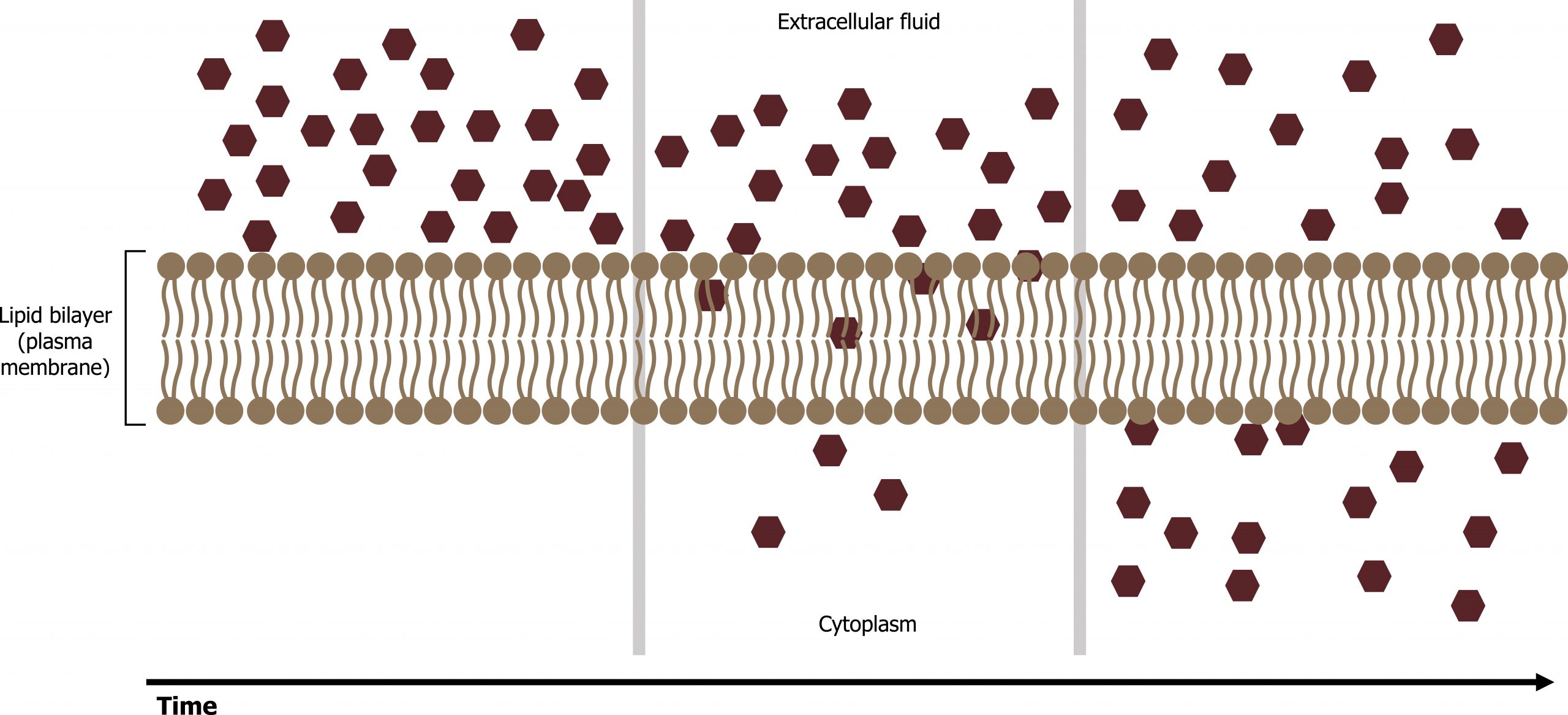
Diffusion is a passive process of transport. A single substance moves from a high concentration to a low concentration area until the concentration is equal across a space (figure 16.4). Materials move within the cellʼs cytosol by diffusion, and certain materials move through the plasma membrane by diffusion such as lipids and fat-soluble vitamins. Diffusion expends no energy. On the contrary, concentration gradients are a form of potential energy, which dissipates as the gradient is eliminated.
Osmosis
Osmosis is the movement of water through a semipermeable membrane according to the waterʼs concentration gradient across the membrane, which is inversely proportional to the solute’s concentration. While diffusion transports material across membranes and within cells, osmosis transports only water across a membrane, and the membrane limits the solute’s diffusion in the water (figure 16.5). Not surprisingly, the aquaporins that facilitate water movement play a large role in osmosis, most prominently in red blood cells and the membranes of kidney tubules. In osmosis, water always moves from an area of higher water concentration to one of lower concentration.
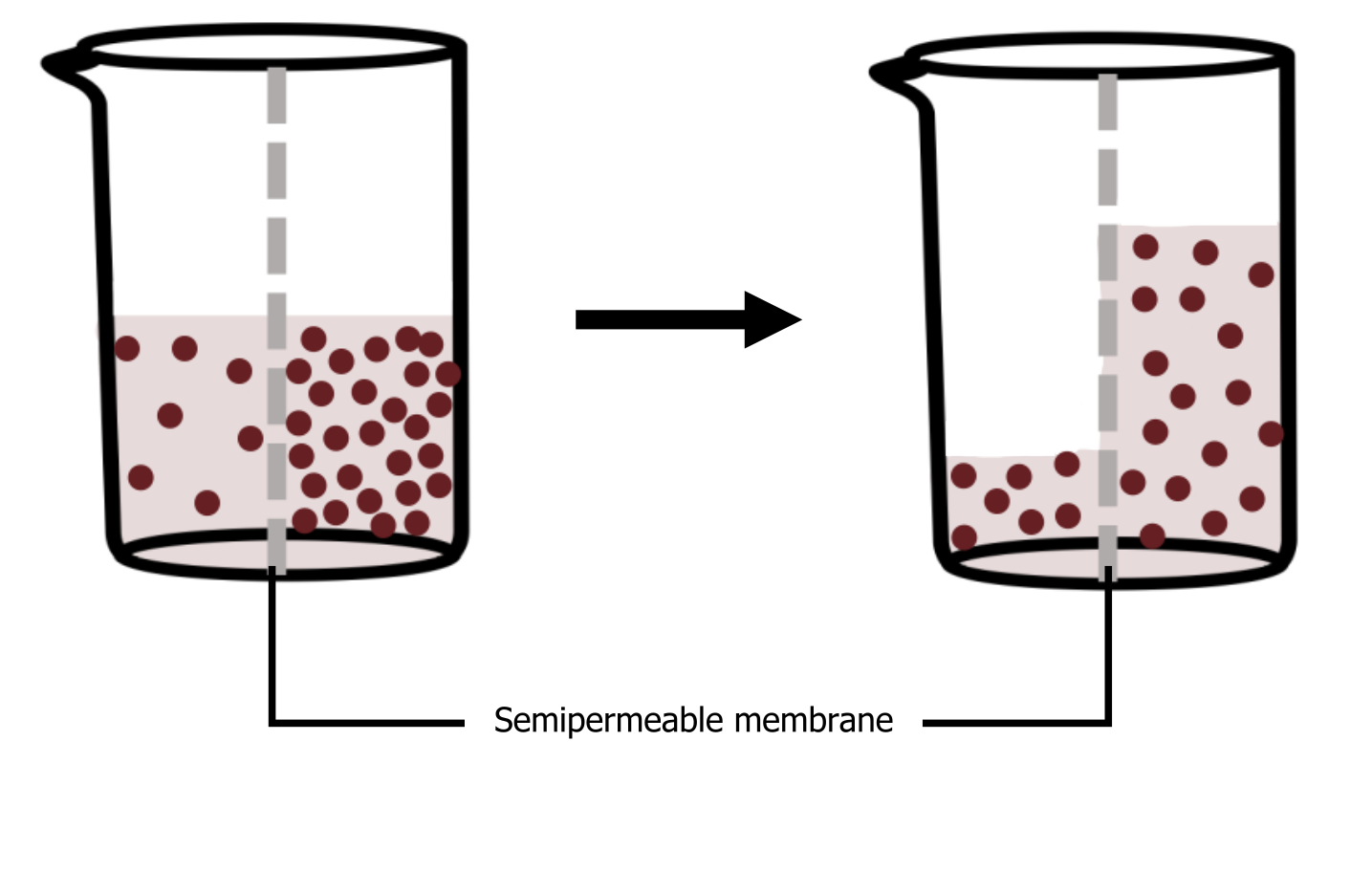
Tonicity
Tonicity describes how an extracellular solution can change a cellʼs volume by affecting osmosis. A solutionʼs tonicity often directly correlates with the solutionʼs osmolarity. Osmolarity describes the solutionʼs total solute concentration.
- A solution with low osmolarity has a greater number of water molecules relative to the number of solute particles.
- A solution with high osmolarity has fewer water molecules with respect to solute particles.
In a situation in which a membrane, permeable to water though not to the solute, separates two different osmolarities, water will move from the membraneʼs side with lower osmolarity (and more water) to the side with higher osmolarity (and less water).
Hypotonic solutions
In a hypotonic situation, the extracellular fluid has lower osmolarity than the fluid inside the cell, and water enters the cell. It also means that the extracellular fluid has a higher water concentration in the solution than does the cell. In this situation, water will follow its concentration gradient and enter the cell.
Hypertonic solutions
As for a hypertonic solution, the prefix “hyper” refers to the extracellular fluid having a higher osmolarity than the cellʼs cytoplasm; therefore, the fluid contains less water than the cell does. Because the cell has a relatively higher water concentration, water will leave the cell.
Isotonic solutions
In an isotonic solution, the extracellular fluid has the same osmolarity as the cell. If the cellʼs osmolarity matches that of the extracellular fluid, there will be no net movement of water into or out of the cell, although water will still move in and out. Osmotic pressure changes red blood cellsʼ shape in hypertonic, isotonic, and hypotonic solutions (figure 16.6).
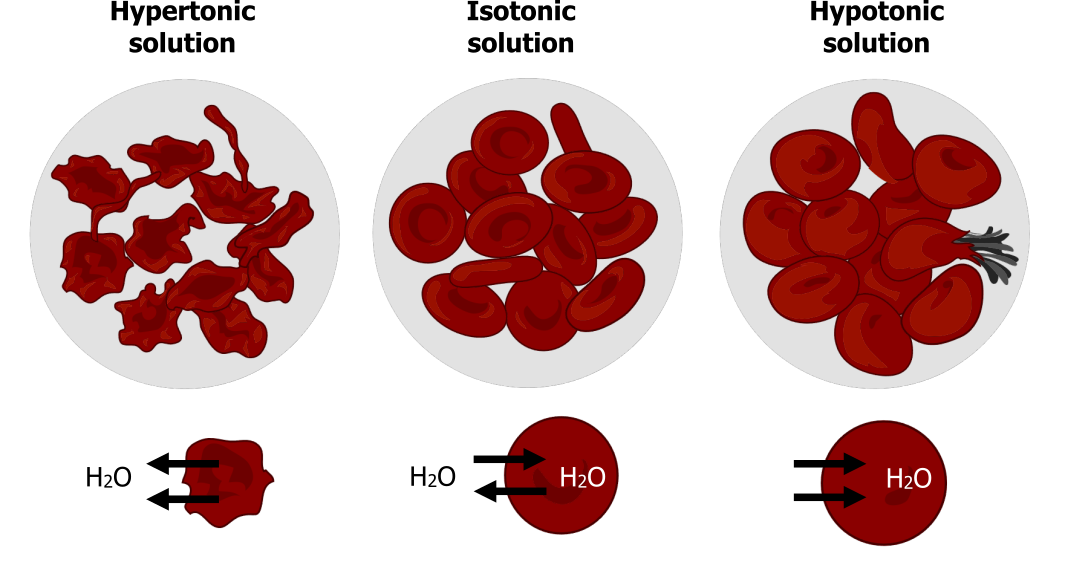
Factors that affect diffusion
Molecules move constantly in a random manner, at a rate that depends on their mass, their environment, and the amount of thermal energy they possess, which in turn is a function of temperature. While diffusion will go forward in the presence of a substanceʼs concentration gradient, several factors affect the diffusion rate:
- Extent of the concentration gradient: The greater the difference in concentration, the more rapid the diffusion. The closer the distribution of the material gets to equilibrium, the slower the diffusion rate.
- Mass of the molecules diffusing: Heavier molecules move more slowly; therefore, they diffuse more slowly. The reverse is true for lighter molecules.
- Temperature: Higher temperatures increase the energy and therefore the moleculesʼ movement, increasing the diffusion rate. Lower temperatures decrease the moleculesʼ energy, thus decreasing the diffusion rate.
- Solvent density: As the density of a solvent increases, the diffusion rate decreases. The molecules slow down because they have a more difficult time passing through the denser medium.
- Solubility: Nonpolar or lipid-soluble materials pass through plasma membranes more easily than polar materials, allowing for a faster diffusion rate.
- Surface area and plasma membrane thickness: Increased surface area increases the diffusion rate, whereas a thicker membrane reduces it.
- Distance traveled: The greater the distance that a substance must travel, the slower the diffusion rate.
Facilitated transport (diffusion)
In facilitated transport, or facilitated diffusion, materials diffuse across the plasma membrane with the help of membrane proteins. A concentration gradient exists that would allow these materials to diffuse into the cell without expending cellular energy. However, these materials are polar molecules or ions that the cell membraneʼs hydrophobic parts repel. Facilitated transport proteins shield these materials from the membraneʼs repulsive force, allowing them to diffuse into the cell.
Ion channels
Channels are specific for the transported substance. Channel proteins have hydrophilic domains exposed to the intracellular and extracellular fluids. In addition, they have a hydrophilic channel through their core that provides a hydrated opening through the membrane layers (figure 16.7).
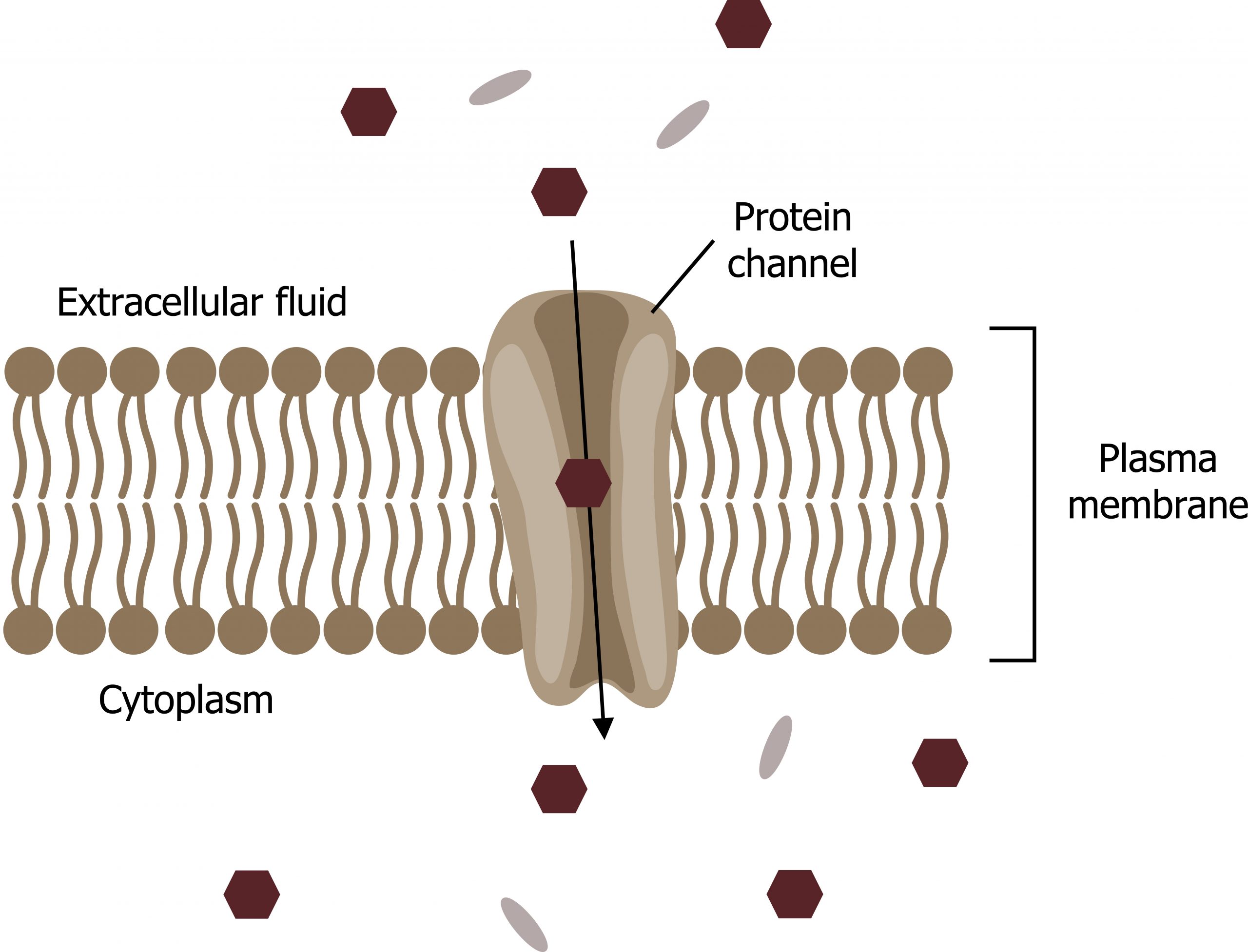
Channel proteins are either open at all times or they are “gated,” which controls the channelʼs opening. The gating can be controlled by volatage, ligand (such as ATP), or mechanical stimulus. When a particular ion attaches to the channel protein, it may control the opening, or other mechanisms or substances may be involved.
In some tissues, sodium and chloride ions pass freely through open channels, whereas in other tissues a gate must open to allow passage. Cells involved in transmitting electrical impulses, such as nerve and muscle cells, have gated channels for sodium, potassium, and calcium in their membranes. Opening and closing these channels changes the relative concentrations on opposing sides of the membrane of these ions, resulting in facilitating electrical transmission along membranes (in the case of nerve cells) or in muscle contraction (in the case of muscle cells).
Carrier proteins
Another type of protein embedded in the plasma membrane is a carrier protein. This aptly named protein binds a substance and, thus triggers a change of its own shape, moving the bound molecule from the cellʼs outside to its interior (figure 16.8).
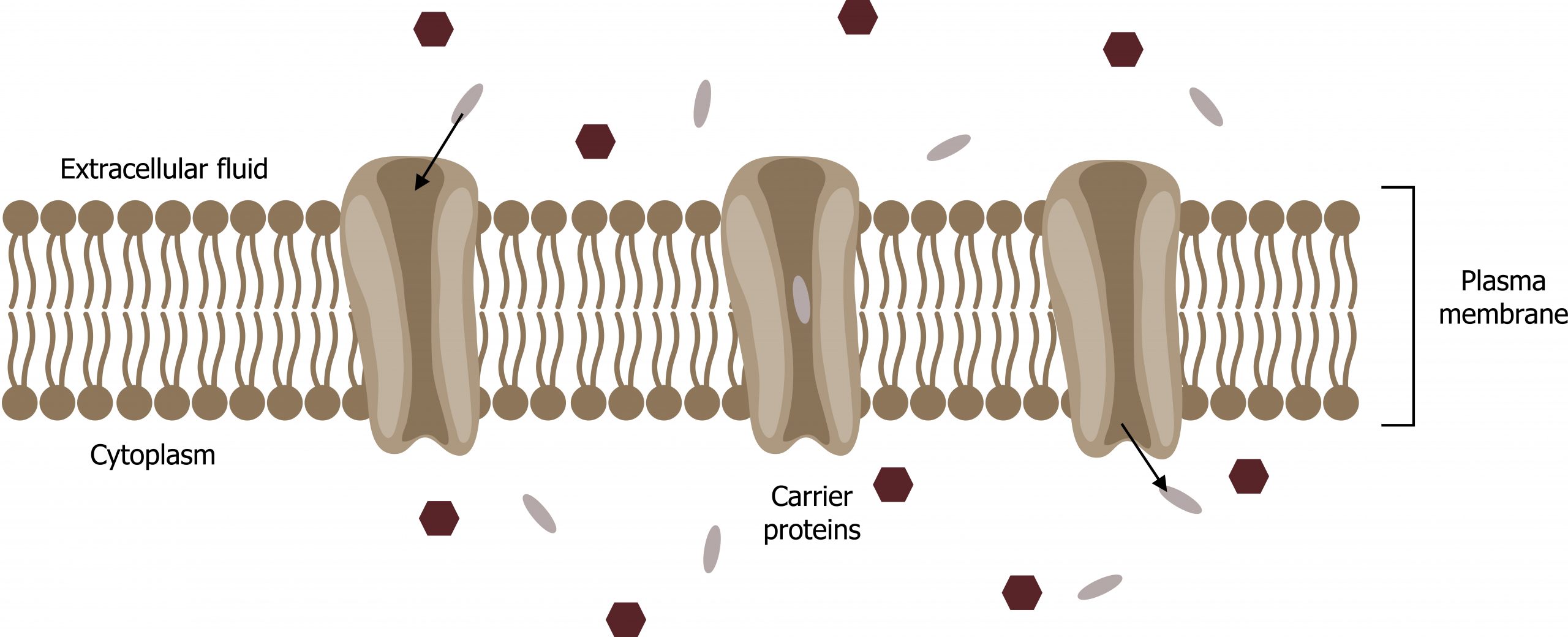
Depending on the gradient, the material may move in the opposite direction. Carrier proteins are typically specific for a single substance. This selectivity adds to the plasma membraneʼs overall selectivity. One group of carrier proteins, glucose transport proteins, or GLUTs, are involved in transporting glucose and other hexose sugars through plasma membranes within the body.
Channel and carrier proteins transport material at different rates. Channel proteins transport much more quickly than carrier proteins. Channel proteins facilitate diffusion at a rate of tens of millions of molecules per second, whereas carrier proteins work at a rate of a thousand to a million molecules per second.
16.2 References and resources
Text
Clark, M. A. Biology, 2nd ed. Houston, TX: OpenStax College, Rice University, 2018, Chapter 4: Cell Structure, Chapter 5: Structure and Function of the Plasma Membranes.
Karp, G., and J. G. Patton. Cell and Molecular Biology: Concepts and Experiments, 7th ed. Hoboken, NJ: John Wiley, 2013, Chapter 4: The Structure and Function of the Plasma Membrane.
Le, T., and V. Bhushan. First Aid for the USMLE Step 1, 29th ed. New York: McGraw Hill Education, 2018, 49.
Figures
Grey, Kindred, Figure 16.4 Diffusion across the plasma membrane. 2021. https://archive.org/details/16.4_20210926. CC BY 4.0. Added ion channel by Léa Lortal from the Noun Project.
Grey, Kindred, Figure 16.5 Illustration of osmosis. In the diagram, the solute cannot pass through the selectively permeable membrane, but the water can. 2021. https://archive.org/details/16.5_20210926. CC BY 4.0.
Grey, Kindred, Figure 16.6 Comparison of red blood cell morphology in isotonic, hypertonic and hypotonic solutions. 2021. https://archive.org/details/16.6_20210926. CC BY 4.0. Added Osmotic pressure on blood cells diagram by LadyofHats. Public domain. From Wikimedia Commons.
Lieberman M, Peet A. Figure 16.7 Protein channel. Adapted under Fair Use from Marks’ Basic Medical Biochemistry. 5th Ed. pp 175 Figure 10.7 Common types of transport mechanisms for human cells. 2017. Added Cell membrane detailed diagram blank by LadyofHats. Public domain. From Wikimedia Commons. Added ion channel by Léa Lortal from the Noun Project.
Lieberman M, Peet A. Figure 16.8 Carrier proteins… Adapted under Fair Use from Marks’ Basic Medical Biochemistry. 5th Ed. pp 175 Figure 10.7 Common types of transport mechanisms for human cells. 2017. Added Cell membrane detailed diagram blank by LadyofHats. Public domain. From Wikimedia Commons. Added ion channel by Léa Lortal from the Noun Project.
16.3 Active Transport
Active transport mechanisms require the cellʼs energy, usually in the form of adenosine triphosphate (ATP). If a substance must move into the cell against its concentration gradient — that is, if the substanceʼs concentration inside the cell is greater than its concentration in the extracellular fluid (and vice versa) — the cell must use energy to move the substance.
Electrochemical gradient
We have discussed simple concentration gradients — a substanceʼs differential concentrations across a space or a membrane — but in living systems, gradients are more complex. Because ions move into and out of cells and because cells contain proteins that do not move across the membrane and are mostly negatively charged, there is also an electrical gradient, a difference of charge, across the plasma membrane.
The interior of living cells is electrically negative with respect to the extracellular fluid in which they are bathed, and at the same time, cells have higher concentrations of potassium (K+) and lower concentrations of sodium (Na+) than the extracellular fluid. Thus in a living cell, the concentration gradient of Na+ tends to drive it into the cell, and its electrical gradient (a positive ion) also drives it inward to the negatively charged interior. However, the situation is more complex for other elements such as potassium. The electrical gradient of K+, a positive ion, also drives it into the cell, but the concentration gradient of K+ drives K+ out of the cell (figure 16.9). We call the combined concentration gradient and electrical charge that affects an ion its electrochemical gradient.
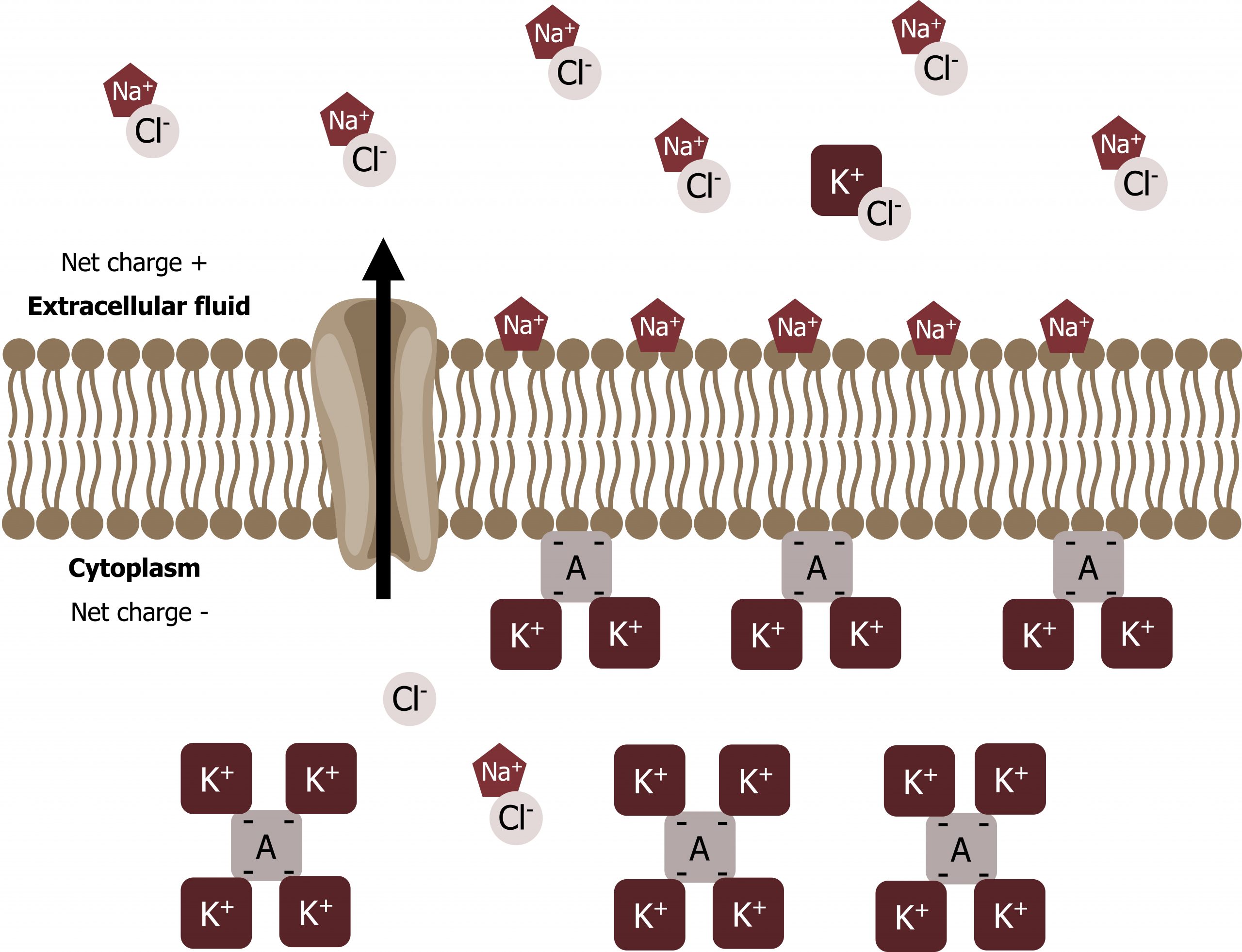
Moving against a gradient
Two mechanisms exist for transporting small molecular weight material and small molecules:
- Primary active transport moves ions across a membrane and creates a difference in charge across that membrane, which is directly dependent on ATP.
- Secondary active transport does not directly require ATP; instead, it is the movement of material due to the electrochemical gradient established by primary active transport.
Carrier proteins for active transport
An important membrane adaptation for active transport is the presence of specific carrier proteins or pumps to facilitate movement. There are three protein types or transporters (figure 16.10).
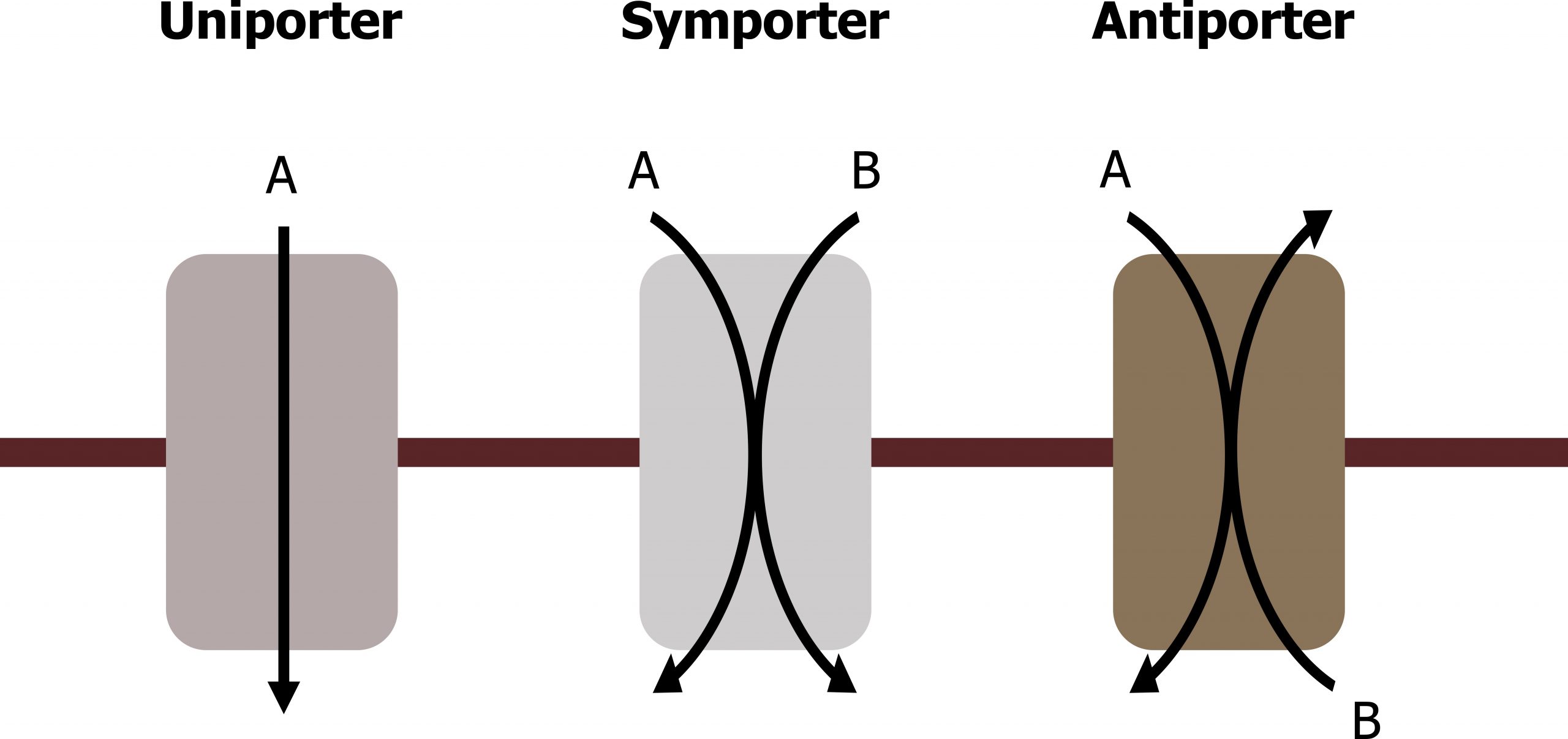
- A uniporter carries one specific ion or molecule.
- A symporter carries two different ions or molecules, both in the same direction.
- An antiporter also carries two different ions or molecules, but in different directions.
All of these transporters can also transport small, uncharged organic molecules like glucose. These three types of carrier proteins are also in facilitated diffusion, but they do not require ATP to work in that process.
Primary active transport
The primary active transport that functions with the active transport of sodium and potassium allows secondary active transport to occur. The second transport method is still active because it depends on using energy as does primary transport (figure 16.11).
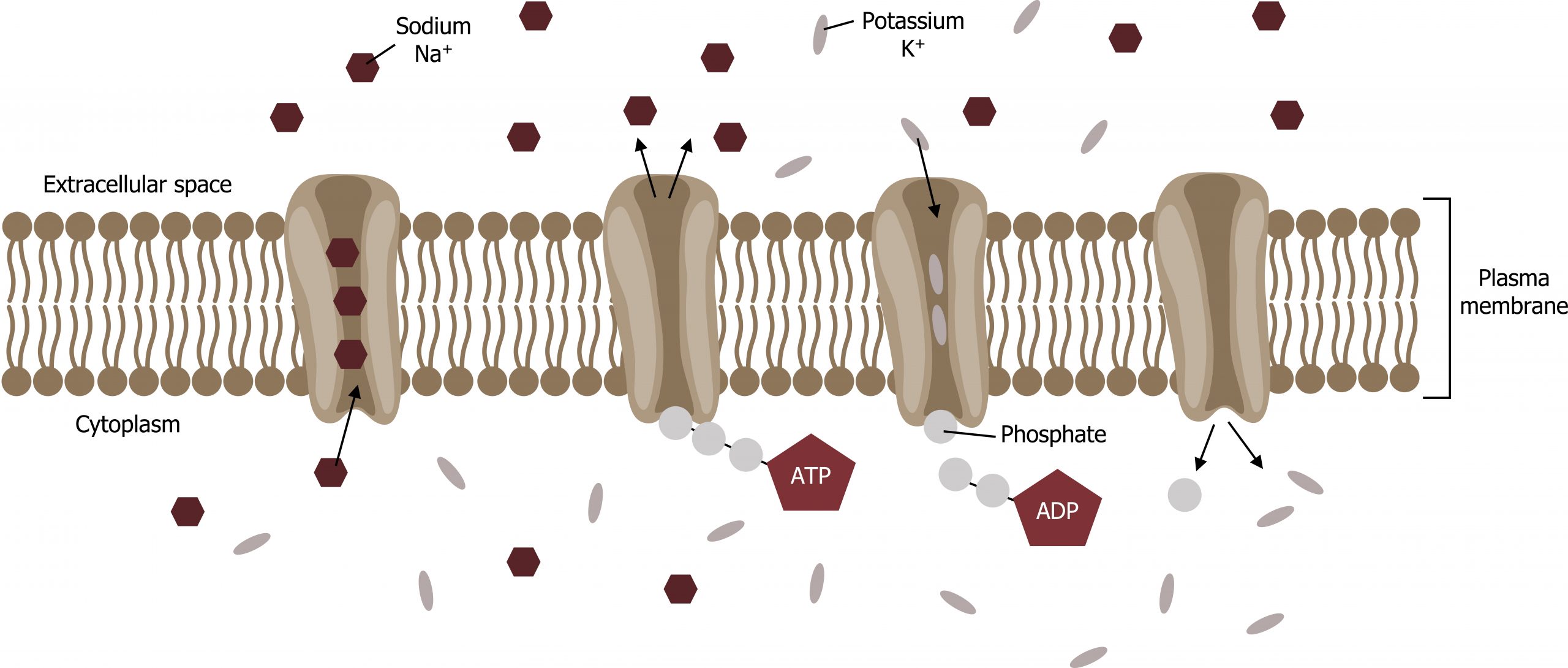
One of the most important pumps in animal cells is the sodium-potassium pump (Na+-K+ ATPase), which maintains the electrochemical gradient (and the correct concentrations of Na+ and K+) in living cells. The sodium-potassium pump moves K+ into the cell while moving Na+ out at the same time, at a ratio of three Na+ for every two K+ ions moved in. The Na+-K+ ATPase exists in two forms, depending on its orientation to the cellʼs interior or exterior and its affinity for either sodium or potassium ions. The process consists of the following six steps.
- With the protein oriented toward the cellʼs interior, the carrier has a high affinity for sodium ions. Three ions bind to the protein.
- The protein carrier hydrolyzes ATP, and a low-energy phosphate group attaches to it.
- As a result, the carrier changes shape and reorients itself toward the membraneʼs exterior. The proteinʼs affinity for sodium decreases, and the three sodium ions leave the carrier.
- The shape change increases the carrierʼs affinity for potassium ions, and two such ions attach to the protein.
- With the phosphate group removed and potassium ions attached, the carrier protein repositions itself toward the cellʼs interior.
- The carrier protein, in its new configuration, has a decreased affinity for potassium, and the two ions move into the cytoplasm. The protein now has a higher affinity for sodium ions, and the process starts again.
Secondary active transport (cotransport)
Secondary active transport brings sodium ions, and possibly other compounds, into the cell. As sodium ion concentrations build up outside of the plasma membrane because of the primary active transport process, this creates an electrochemical gradient. If a channel protein exists and is open, the sodium ions will pull through the membrane. This movement transports other substances that can attach themselves to the transport protein through the membrane. Many amino acids, as well as glucose, enter a cell this way.
16.3 References and resources
Text
Clark, M. A. Biology, 2nd ed. Houston, TX: OpenStax College, Rice University, 2018, Chapter 4: Cell Structure, Chapter 5: Structure and Function of the Plasma Membranes.
Karp, G., and J. G. Patton. Cell and Molecular Biology: Concepts and Experiments, 7th ed. Hoboken, NJ: John Wiley, 2013, Chapter 4: The Structure and Function of the Plasma Membrane.
Le, T., and V. Bhushan. First Aid for the USMLE Step 1, 29th ed. New York: McGraw Hill Education, 2018, 49.
Figures
Grey, Kindred, Figure 16.9 Electrochemical gradients. 2021. https://archive.org/details/16.9_20210926. CC BY 4.0. Added Cell membrane detailed diagram blank by LadyofHats. Public domain. From Wikimedia Commons. Added ion channel by Léa Lortal from the Noun Project.
Grey, Kindred, Figure 16.10 Different types of carrier proteins for active transport. 2021. https://archive.org/details/16.10. CC BY 4.0.
Lieberman M, Peet A. Figure 16.11 Primary active transport. Adapted under Fair Use from Marks’ Basic Medical Biochemistry. 5th Ed. pp 175 Figure 10.10 Active transport by Na+,K+-ATPase. 2017. Added Cell membrane detailed diagram blank by LadyofHats. Public domain. From Wikimedia Commons. Added ion channel by Léa Lortal from the Noun Project.