7 Pentose Phosphate Pathway (PPP), Purine and Pyrimidine Metabolism
Learning Objectives
- Describe the role of NADPH produced by the pentose phosphate pathway in metabolism and regulation of glucose 6-phosphate dehydrogenase.
- Determine the utility of the oxidative and nonoxidative portions of the pentose phosphate pathway (PPP) and how these pathways interface with glycolysis.
- Describe the amino acid composition of glutathione (GSH) and understand the role of GSH in attenuating oxidative damage.
- Describe how the pentose phosphate pathway and the process of DNA replication interface with the biosynthesis of purine and pyrimidine nucleotides.
- Evaluate the central role of 5-phosphoribosyl-1-pyrophosphate (PRPP) in nucleotide metabolism.
- Describe the purine salvage pathway, specifically the reaction catalyzed by hypoxanthine-guanine phosphoribosyltransferase (HGPRT).
- Identify the key regulatory steps in both purine and pyrimidine synthesis, and evaluate flux through each pathway depending on levels of allosteric activators and inhibitors.
- Describe conditions that lead to elevated orotic acid, and interpret urine orotic acid concentration for the diagnosis of defects of the urea cycle or pyrimidine biosynthesis.
About this Chapter
As we have seen previously, glucose can be diverted to several different pathways depending on metabolic needs. One of these pathways is the pentose phosphate pathway, which plays an integral role in producing both NADPH and the five-carbon sugar ribose. NADPH provides the cell with an energy source for reductive biosynthesis and detoxification of free radicals, while ribose is an essential component in the synthesis of both purine and pyrimidine nucleotides. Aberrations (increases or decreases) in either of these metabolic pathways, PPP or nucleotide synthesis, can result in the common clinical presentations of anemia, jaundice, or gout.
7.1 Pentose Phosphate Pathway
The pentose phosphate pathway (PPP — also known as the hexose monosphosphate shunt) is a cytosolic pathway that interfaces with glycolysis. In this pathway, no ATP is directly produced from the oxidation of glucose 6-phosphate; instead the oxidative portion of the PPP is coupled to the production of NADPH. In addition to generating NADPH, which is essential for detoxification reactions and fatty acid synthesis, it also produces five-carbon sugars required for nucleotide synthesis.
Oxidative and nonoxidative functions
There are two parts of the pathway that are distinct and can be regulated independently. The first phase, or oxidative phase, consists of two irreversible oxidations that produce NADPH. As noted above, NADPH is required for reductive detoxification and fatty acid synthesis. (NADPH is not oxidized in the ETC.) In the red blood cell, this is extremely important as the PPP pathway provides the only source of NADPH. NADPH is essential to maintain sufficient levels of reduced glutathione in the red blood cell. Glutathione is a tripeptide commonly used in tissues to detoxify free radicals and reduce cellular oxidation.
The nonoxidative phase of the pathway allows for the conversion of ribulose 5-phosphate into ribose 5-phosphate, which is needed for nucleotide synthesis (figure 7.1). All of these interconversions in the nonoxidative pathway are reversible and use the enzymes transketolase or transaldolase to move two-carbon or three-carbon units on to other sugar moieties to generate a variety of sugar intermediates. Transketolase requires thiamine pyrophosphate (TPP) as a cofactor. This is of clinical relevance as TPP levels can be measured by addressing the activity of transketolase in a blood sample. A reduction in transketolase activity is an indicator of a thiamine deficiency.
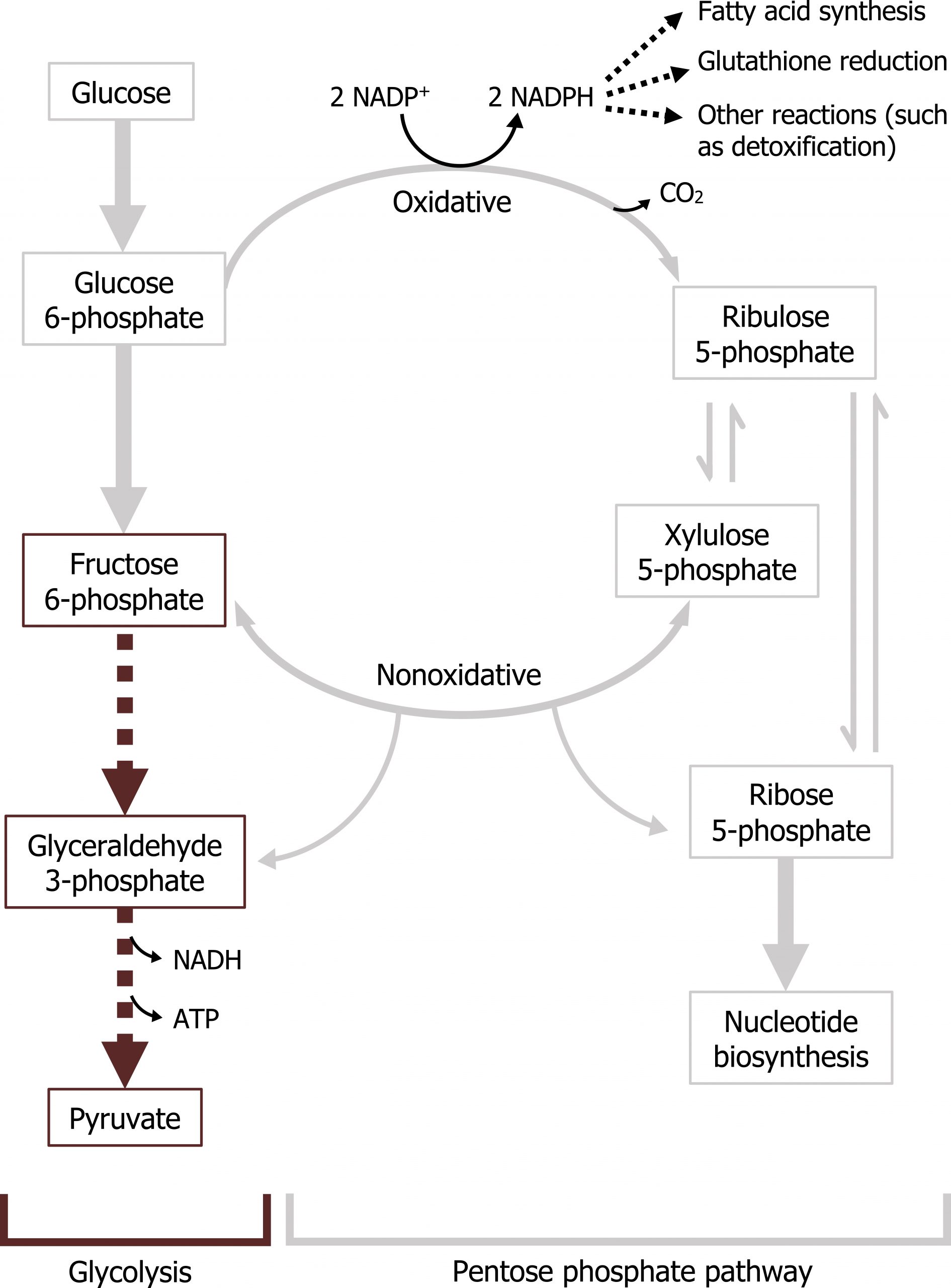
Any compounds unused by the nonoxidative pathway will eventually be converted to fructose 6-phosphate or glyceraldehyde 3-phosphate, both of which will re-enter the glycolytic pathway (figures 7.1 and 7.2).
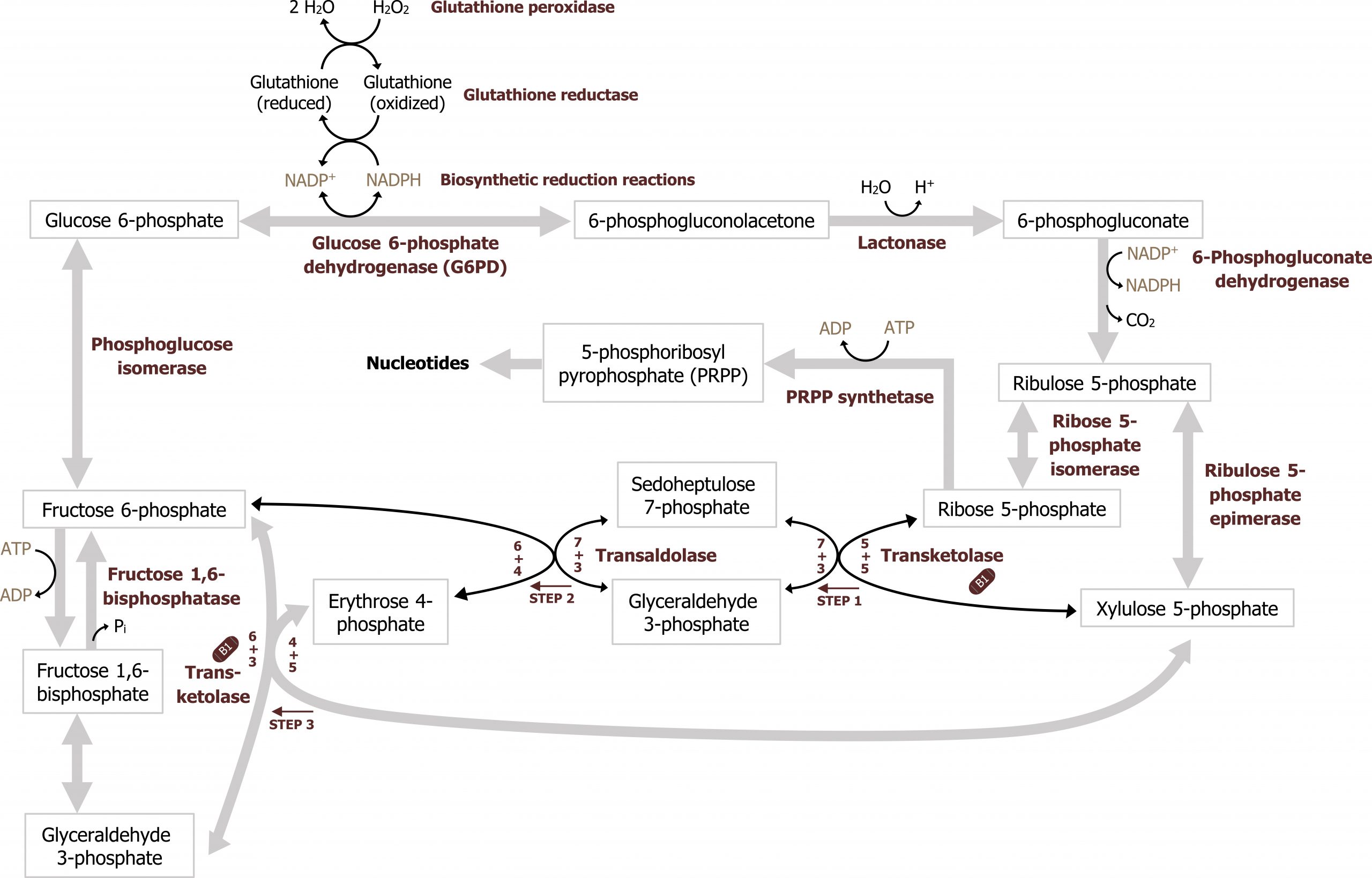
Regulation of the pentose phosphate pathway
The key regulatory enzyme for the pentose phosphate pathway is within the oxidative portion. Glucose 6-phosphate dehydrogenase oxidizes glucose 6-phosphate to 6-phosphogluconolactone, and is regulated by negative feedback. In this two-step reaction NADPH is also produced, and high levels of NADPH will inhibit the activity of glucose 6-phosphate dehydrogenase. This ensures NADPH is only generated as needed by the cell; this is the primary regulatory mechanism within the pathway.
The nonoxidative phase is not regulated; however, in conditions where there is a high demand for nucleotide production (such as in the case for highly proliferative cells), the nonoxidative part of the pathway can function independently of the oxidative phase to produce ribose 5-phosphate from the glycolytic intermediates fructose 6-phosphate and glyceraldehyde 3-phosphate (figure 7.2).
Requirement of the pentose phosphate pathway in RBCs
The two essential products of this pathway are NADPH and ribose 5-phosphate. NADPH is a high-energy compound often used for reductive biosynthesis as it cannot be oxidized in the ETC. It is also used by many tissues to scavenge (and detoxify) reactive oxygen species (ROS) before causing cellular damage. This is especially important in red blood cells; RBCs lack malic enzyme, making this the only pathway that can generate NADPH. A lack of NADPH in RBCs (such as due to a glucose 6-phosphate dehydrogenase deficiency) can cause excessive hemolysis, leading to the clinical presentation of jaundice (figure 7.3).
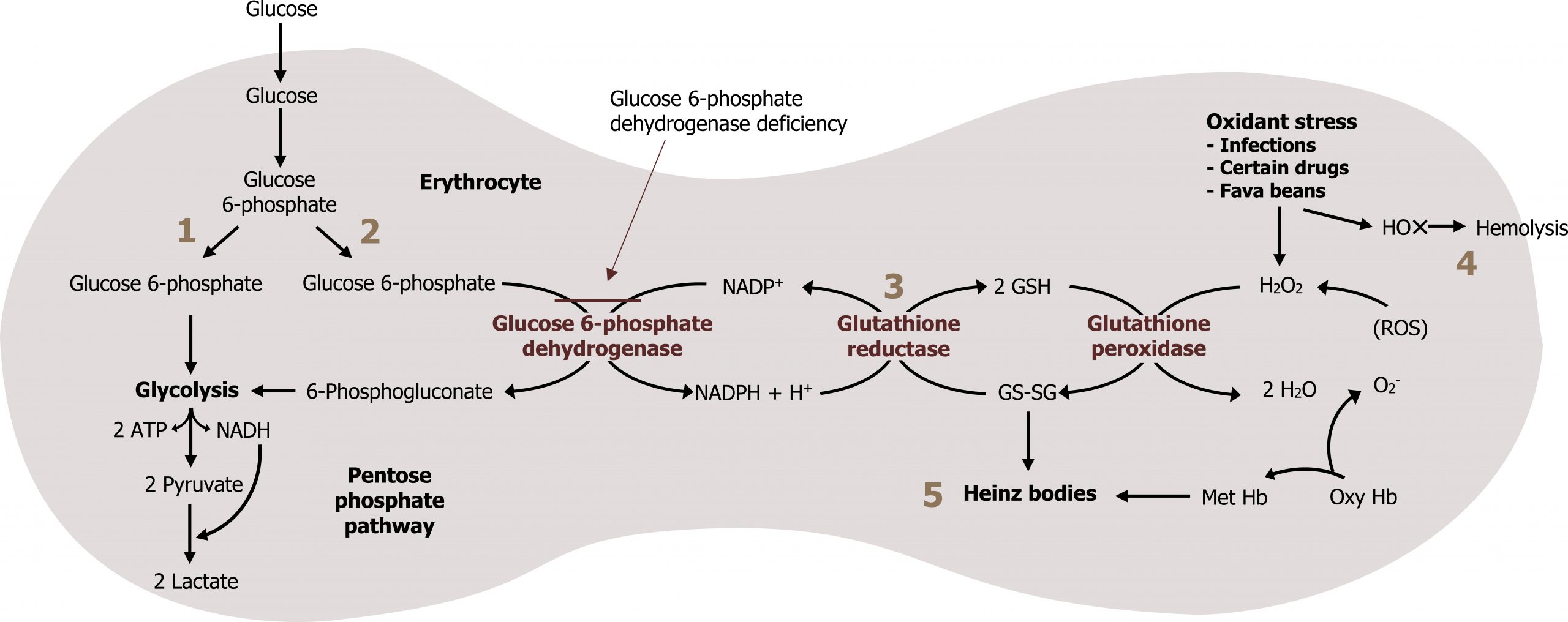
Glutathione (GSH) is a tripeptide compound consisting of glutamate, cysteine, and glycine. It plays a key role in scavenging reactive oxygen species (ROS), which cause both DNA and cellular/protein damage. Reduction of GSH in the red blood cell is done exclusively through a series of oxidation reduction reactions using NADPH. The loss of NADPH in RBCs therefore increases ROS and can lead to hemolysis (figure 7.3).
Summary of pathway regulation
Metabolic pathway | Major regulatory enzyme | Allosteric effectors | Hormonal effects |
---|---|---|---|
Pentose phosphate pathway | Glucose 6-phosphate dehydrogenase | NADPH (-) | None |
Table 7.1: Summary of pathway regulation.
7.1 References and resources
Text
Ferrier, D. R., ed. Lippincott Illustrated Reviews: Biochemistry, 7th ed. Philadelphia: Wolters Kluwer Health/Lippincott Williams & Wilkins, 2017, Chapter 13: Pentose Phosphate Pathway and NAPDH, Chapter 22: Nucleotide Metabolism.
Le, T., and V. Bhushan. First Aid for the USMLE Step 1, 29th ed. New York: McGraw Hill Education, 2018, 35–37, 79.
Lieberman, M., and A. Peet, eds. Marks’ Basic Medical Biochemistry: A Clinical Approach, 5th ed. Philadelphia: Wolters Kluwer Health/Lippincott Williams & Wilkins, 2018, Chapter 27: Pentose Phosphate Pathway, Chapter 39: Purine and Pyrimidine Synthesis.
Figures
Grey, Kindred, Figure 7.2 Pentose pathway and its connection to glycolysis and glutathione synthesis. 2021. https://archive.org/details/7.2_20210926. CC BY 4.0.
Lieberman M, Peet A. Figure 7.1 Overview of the pentose phosphate pathway and its interface with glycolysis. Adapted under Fair Use from Marks’ Basic Medical Biochemistry. 5th Ed. pp 543. Figure 27.1 Overview of the pentose phosphate pathway. 2017.
Lieberman M, Peet A. Figure 7.3 NADPH in the red blood cell as a means of reducing glutathione. Adapted under Fair Use from Marks’ Basic Medical Biochemistry. 5th Ed. pp 549. Figure 27.7 Hemolysis caused by reactive oxygen species (ROS). 2017.
7.2 Nucleotide Synthesis
Nucleotides are the fundamental building blocks essential for the synthesis of DNA and RNA. Each nucleotide contains three functional groups: a sugar, a base, and phosphate (figure 7.4).
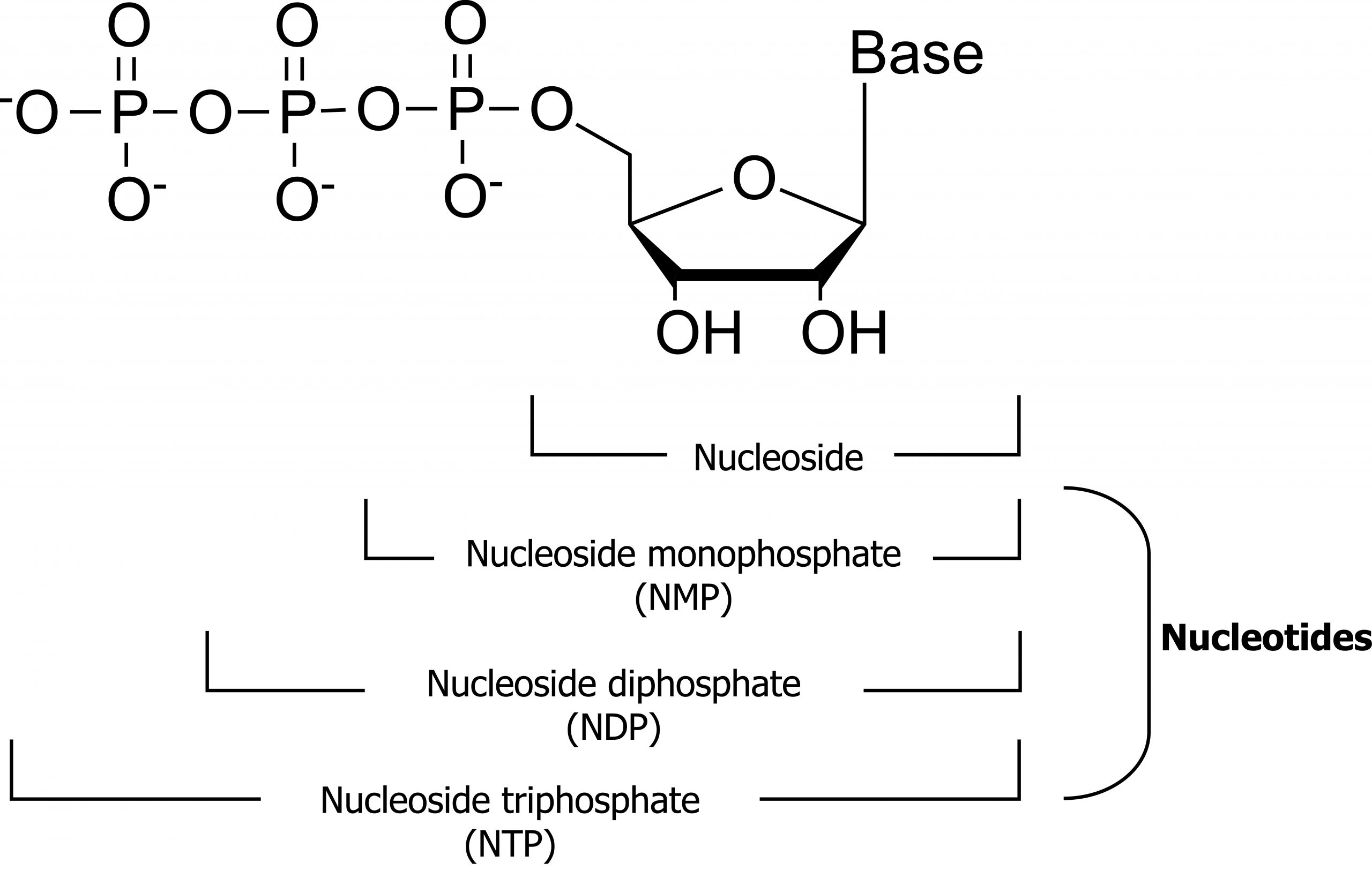
Nucleotides can be divided into two groups: pyrimidines and purines. The family of pyrimidines includes thymine (T), cytosine (C), and uracil (U), which is only incorporated into RNA. These compounds contain a single-ringed nitrogenous base that pairs with a purine nucleotide counterpart. Thymine pairs with adenine forming two hydrogen bonds, in contrast to cytosine, which pairs with guanine to form three hydrogen bonds. Purines, both guanine (G) and adenine (A), are double-ringed structures and more difficult to break down in the body. As such, the salvage pathway for purine metabolism is of importance (figure 7.5).
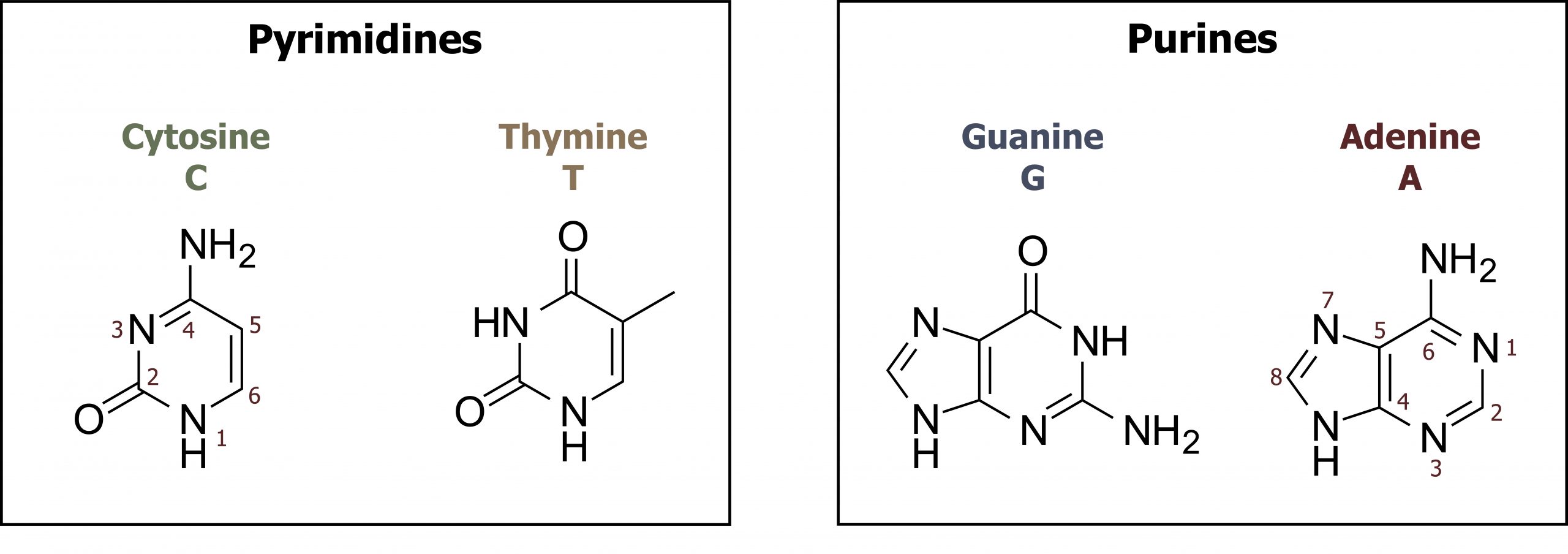
Nucleotide synthesis will be described below, but one of the fundamental requirements of the synthesis of either purines or pyrimidines is the need for a five-carbon sugar (ribose). This sugar is generated through glucose oxidation via the pentose phosphate pathway.
For purines synthesis, the base is synthesized and attached to the sugar, while for pyrimidine synthesis, the sugar group is added after the base is produced. In either case, ribose is the added sugar, and this must be converted to the deoxyribose form before the bases can be used for DNA synthesis.
Conversion of ribose to deoxyribose nucleotides
All bases are synthesized in the ribose form and used directly for transcription. They can be converted to the deoxy form, which is needed for DNA replication. The enzyme, ribonucleotide reductase, converts the diphosphate form of a ribose base to the deoxybase form. The enzyme has two sites for regulation: an enzyme activity site and a substrate specificity site. The enzyme activity site must have ATP/ADP bound for the enzyme to be active, while the substrate specificity site will bind different nucleotides influencing the enzyme substrate preference, therefore altering which base is being acted upon depending on cellular needs.
Generation of 5-phosphoribosyl-1-phosphate (PRPP)
Ribose 5-phosphate is not used directly for either purine or pyrimidine synthesis, rather it is used to synthesize the “active pentose” — 5-phosphoribosyl-1-pyrophosphate (PRPP). The conversion is catalyzed by the enzyme phosphoribosyl-1-pyrophosphate (PRPP) synthase. PRPP is the activated five-carbon sugar used for nucleotide synthesis and provides both the sugar and phosphate group to nucleotides (figure 7.6).
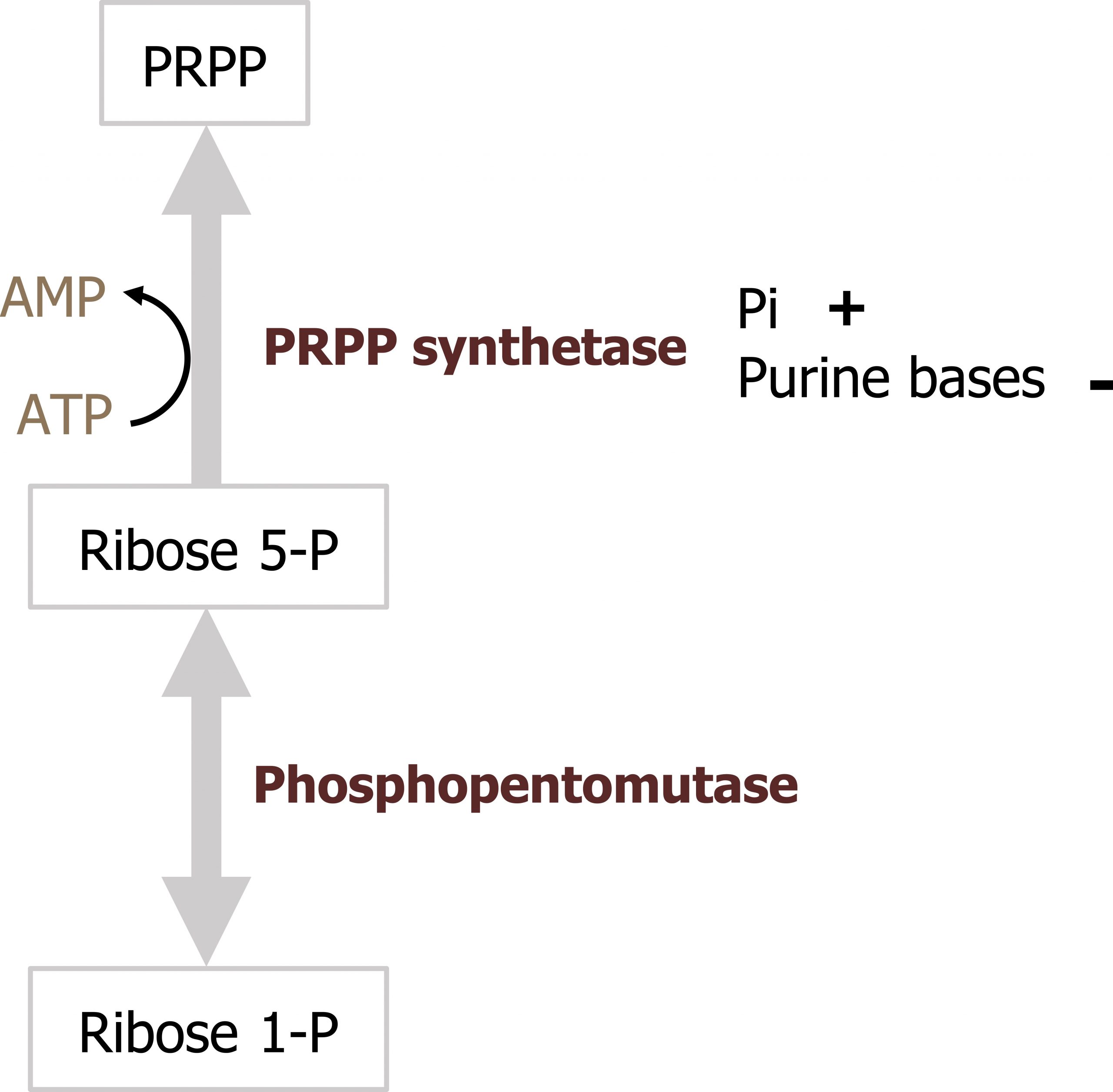
Regulation of PRPP synthase
The enzyme, PRPP synthetase, is activated by Pi (inorganic phosphate) and inhibited by the purine bases adenine and guanine.
Synthesis of purines
Purines are composed of a bicyclic structure that is synthesized from carbon and nitrogen donated from various compounds such as carbon dioxide, glycine, glutamine, aspartate, and tetrahydrofolate (TH4). The synthesis of purines starts with the synthesis of 5ʼphosphoribosylamine from PRPP and glutamine. The enzyme glutamine phosphoribosylpyrophate amidotransferase (GPAT) catalyzes this reaction and is the committed step in purine synthesis (figure 7.7). Synthesis continues for nine additional steps culminating in the synthesis of inosine monophosphate (IMP), which contains the base hypoxanthine. IMP is used to generate both AMP and GMP. The synthesis of both AMP and GMP requires energy in the form of the alternative base (i.e., the synthesis of GMP requires ATP while AMP synthesis requires energy in the form of GTP). The synthesis of AMP and GMP is regulated by feedback inhibition (figures 7.7 and 7.8). This allows for the maintenance of nucleotides in a relative ratio that is required for cellular processes. The generated nucleotide monophosphates can be converted to the di and triphosphate forms by nucleotide specific kinases, which will transfer phosphate groups to maintain a balance of the mono, di, and triphosphate forms.
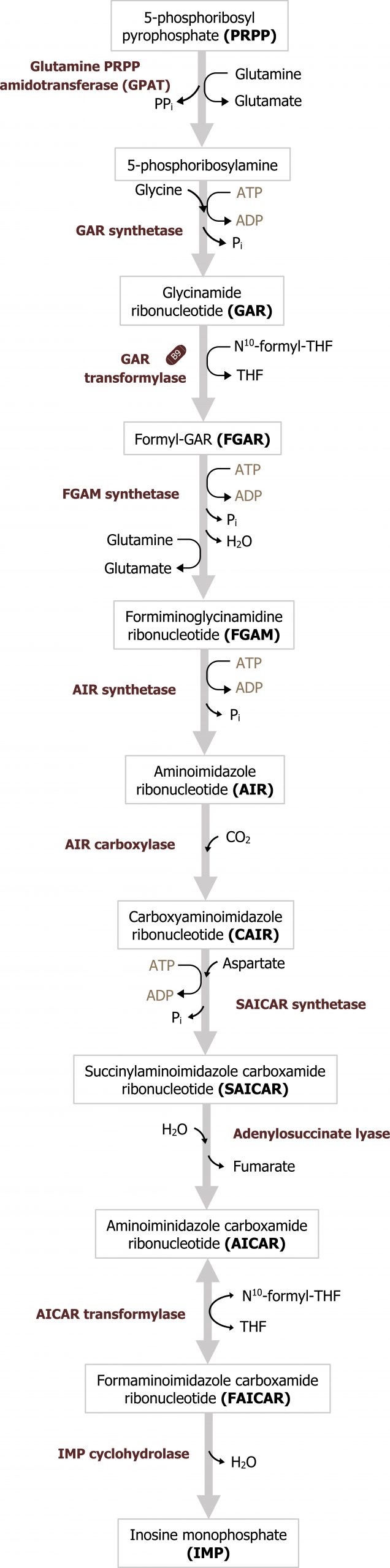
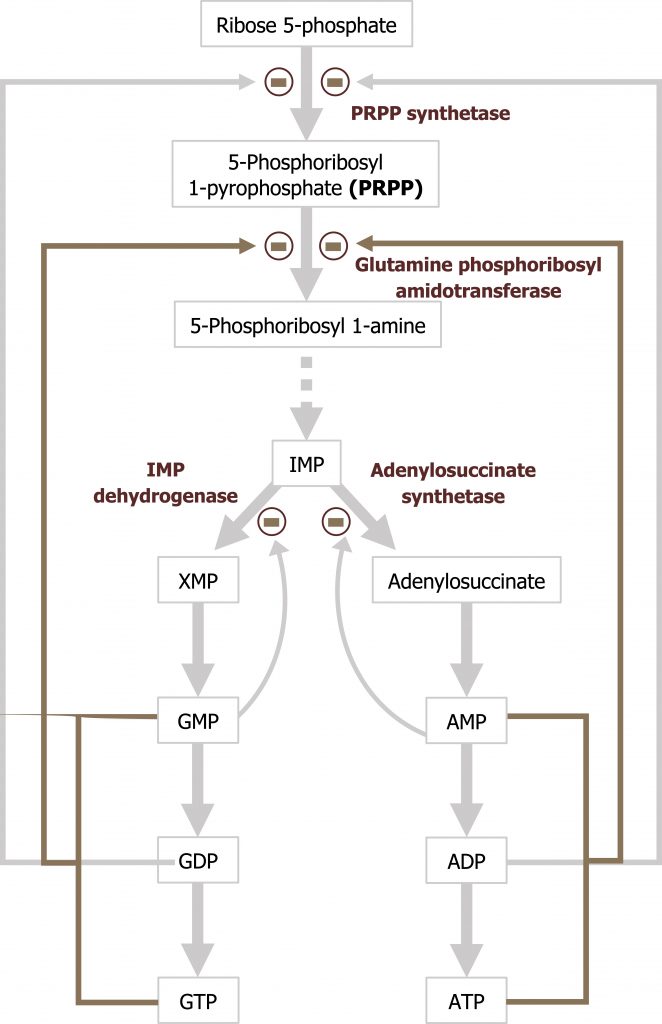
Regulation of purine synthesis
The regulatory enzyme GPAT is allosterically activated by PRPP and inhibited by IMP, AMP, and GMP. All three must be present to inhibit activity of this enzyme.
Degradation of purines
Like amino acids, nucleotides contain nitrogen and must be degraded in a manner that allows for proper nitrogen disposal either through the urea cycle or by the synthesis of a nontoxic compound.
Degradation of dietary nucleotides occurs in the gut, while nucleotides from de novo synthesis are degraded in the liver. The fundamental process involves the dismantling of the sugar, phosphate, and base structure into their own respective units (figure 7.9). In the case of purine degradation, the base is excreted in the form of uric acid. Purine nucleoside phosphorylase converts inosine and guanosine to their respective bases (hypoxanthine and guanine). Finally, xanthine oxidase will oxidize hypoxanthine to xanthine (guanine can be deaminated to xanthine), and xanthine can be further oxidized to uric acid by the same enzyme. Uric acid is excreted in the urine.
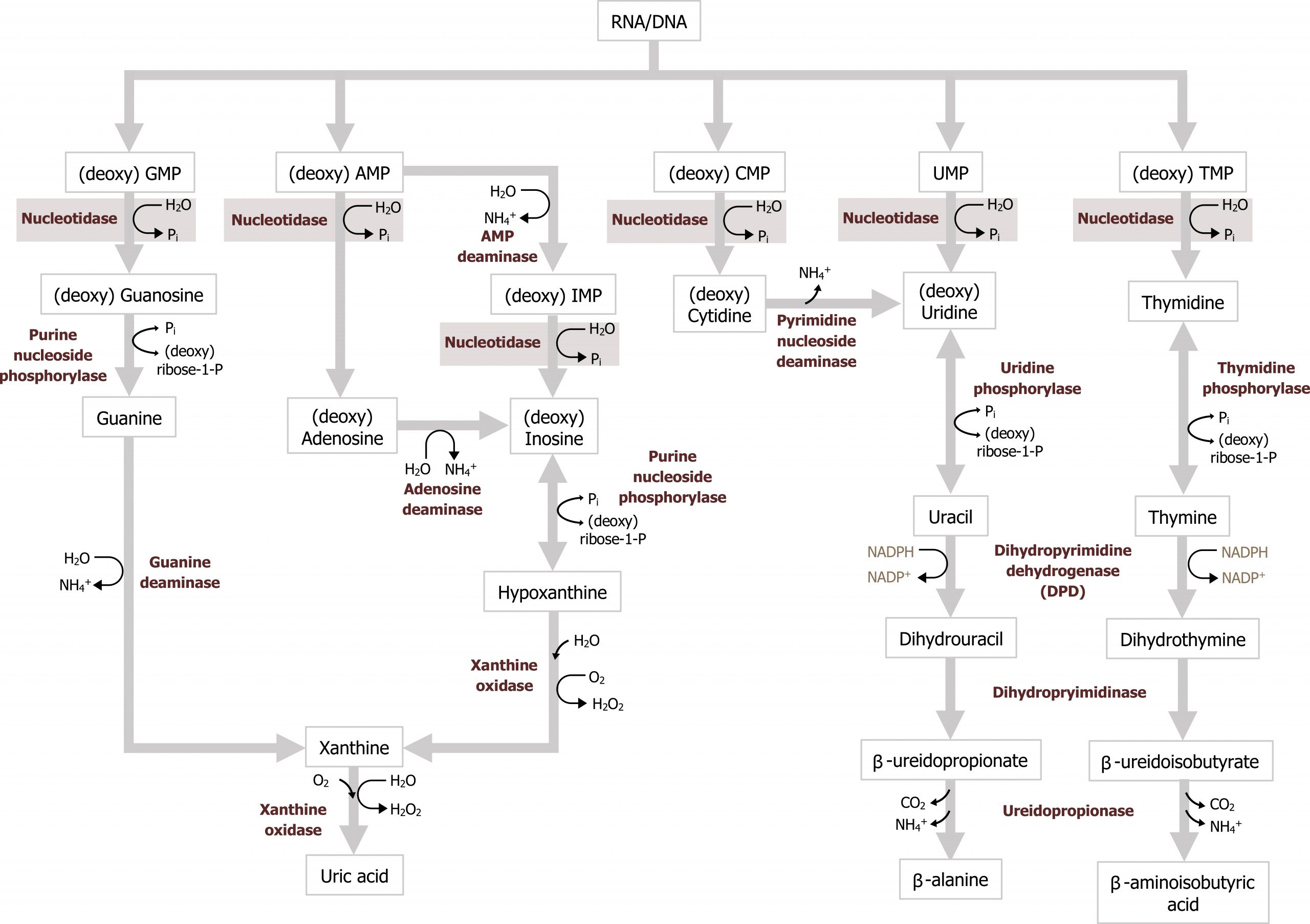
Excess uric acid, hyperuricemia, can cause the precipitation of uric acid crystals in the joints eliciting an inflammatory reaction causing acute pain or gout. The majority of individuals diagnosed with gout present due to underexcretion of uric acid. And this can be caused by the presence of other pathologies, such as lactic acidosis or the use of diuretics. Less common presentations of gout are associated with overproduction of uric acid, which can be caused by increased activity of PRPP synthetase or deficiency in purine recycling enzyme HGPRT caused by Lesch-Nyhan syndrome (figure 7.10).
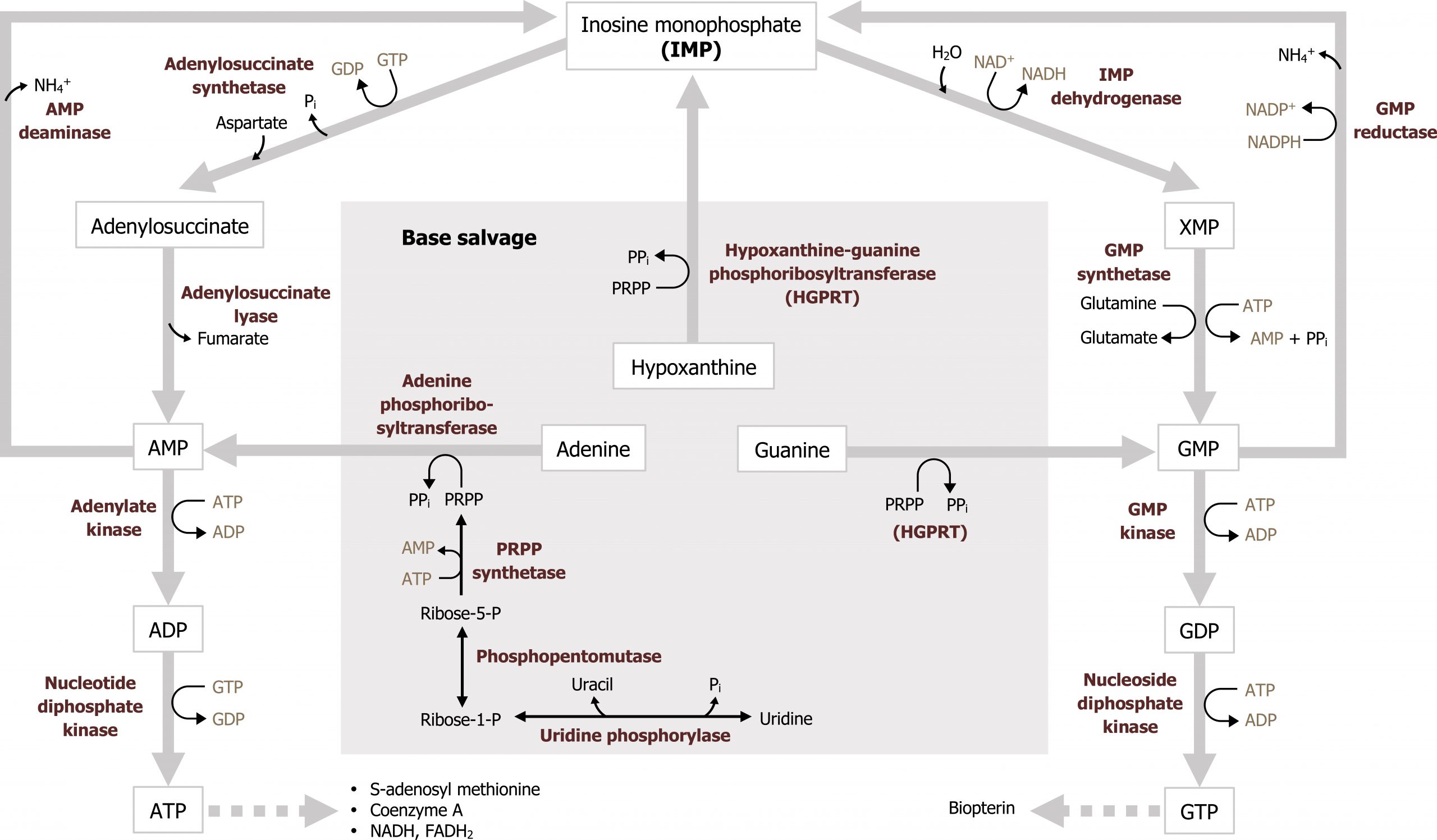
Secondary hyperuricemia is also seen in individuals with myeloproliferative disorders undergoing therapy where there is excess cellular turnover (cell lysis leads to an accumulation of nucleotides) or in cases of Von Gierke disease or fructose intolerance, which increases substrate for PRPP synthesis. Xanthine oxidase inhibitors, such as allopurinol, are used as part of the management of gout.
Salvage of purines
The ability to recycle nucleotides is specifically important in the case of purines as de novo synthesis uses much more ATP than salvage. The degradation product of purine bases is uric acid, which is an insoluble compound, and accumulation can result in several clinical disorders as previously discussed. As such, purine bases can also undergo salvage reaction where bases are recycled and used in a new process. To reduce the amount of uric acid production, purines can be salvaged and reconverted back to their triphosphate form to be reused. There are two primary enzymes involved in the salvage pathway: adenine phosphoribosyltransferase (APRT) and xanthine-guanine phosphoribosyltransferase (HGPRT) (figure 7.10). These enzymes will recombine the base (either adenine, guanine, or hypoxanthine) with PRPP to generate AMP, GMP, or IMP respectively. Adenosine is the only nucleoside that can be rephosphorylated to its monosphosphate form using adenosine kinase (figure 7.11). All other nucleosides must be degraded to their free base before they can be salvaged.
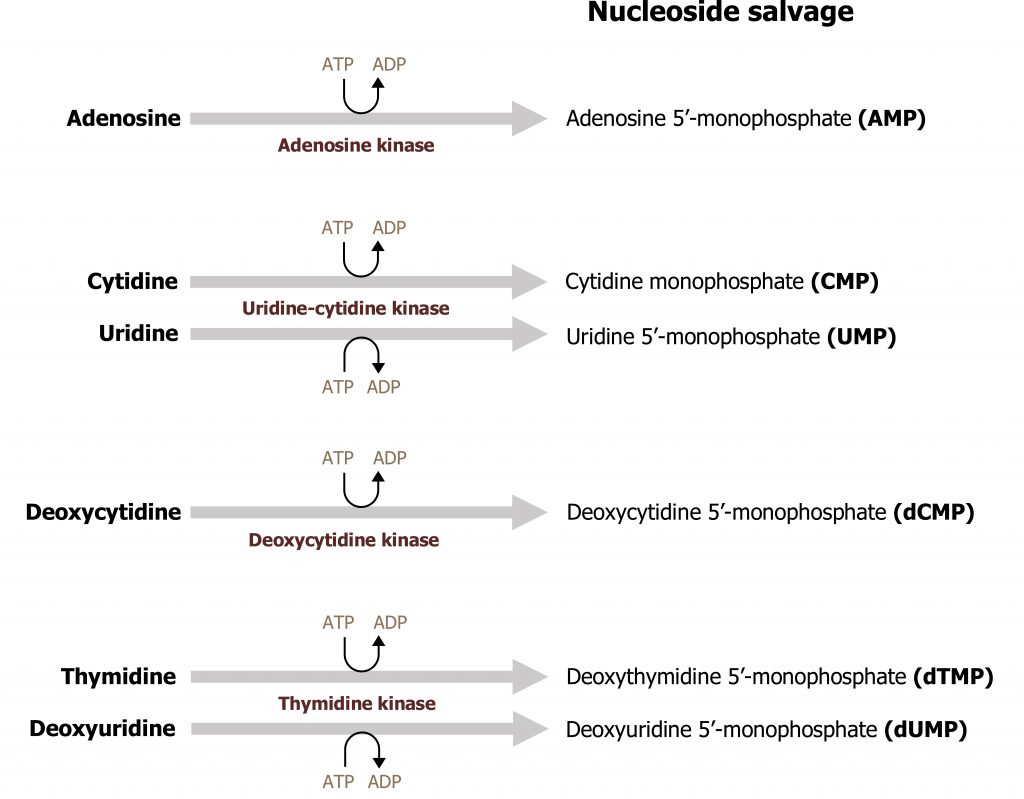
Synthesis of pyrimidines
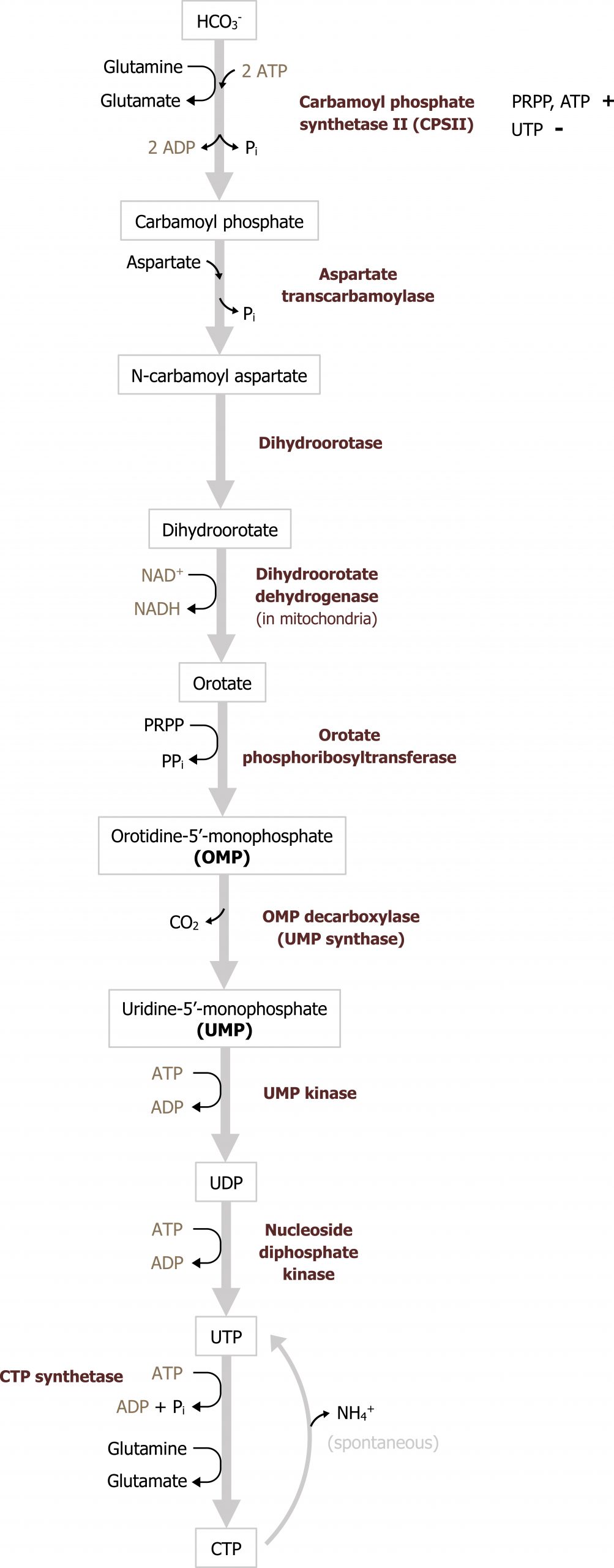
In contrast to purine synthesis, the pyrimidine bases are synthesized before the ribose sugar and phosphate groups are added in the form of PRPP (figure 7.12). The initial step of the pathways involves the synthesis of carbamoyl phosphate from glutamine, carbon dioxide, and 2 ATP. Carbamoyl phosphate synthetase II (CSPII) catalyzes this reaction. (Note there is an analogous enzyme in the mitochondria for the urea cycle termed carbamoyl phosphate synthetase I, which also generates carbamoyl phosphate.) Of clinical importance is the intermediate orotate. Elevations of orotate (orotic acid) are consistent with enzymatic deficiencies in this pathway or urea cycle deficiencies such as a defect in ornithine transcarbamoylase. In the case of a urea cycle deficiency, an excess carbamoyl phosphate can enter pyrimidine synthesis leading to a build up of orotate. Following the synthesis of carbamoyl phosphate, a series of subsequent reactions yield uracil monosphosphate, which is the intermediate of pyrimidine synthesis.
UMP, much like IMP, serves as the intermediate to pyrimidine synthesis and can undergo sequential phosphorylation to form UTP, which can be converted to cytidine (CTP). Alternatively, UMP can be converted to a deoxy form (dUDP) to be used as substrate for the synthesis of thymidine. The conversion of dUDP to dTMP is catalyzed by thymidylate synthase, which requires folate (N5,N10 methylene tetrahydrofolate) as a methyl and hydrogen donor to complete this conversion (figure 7.13).
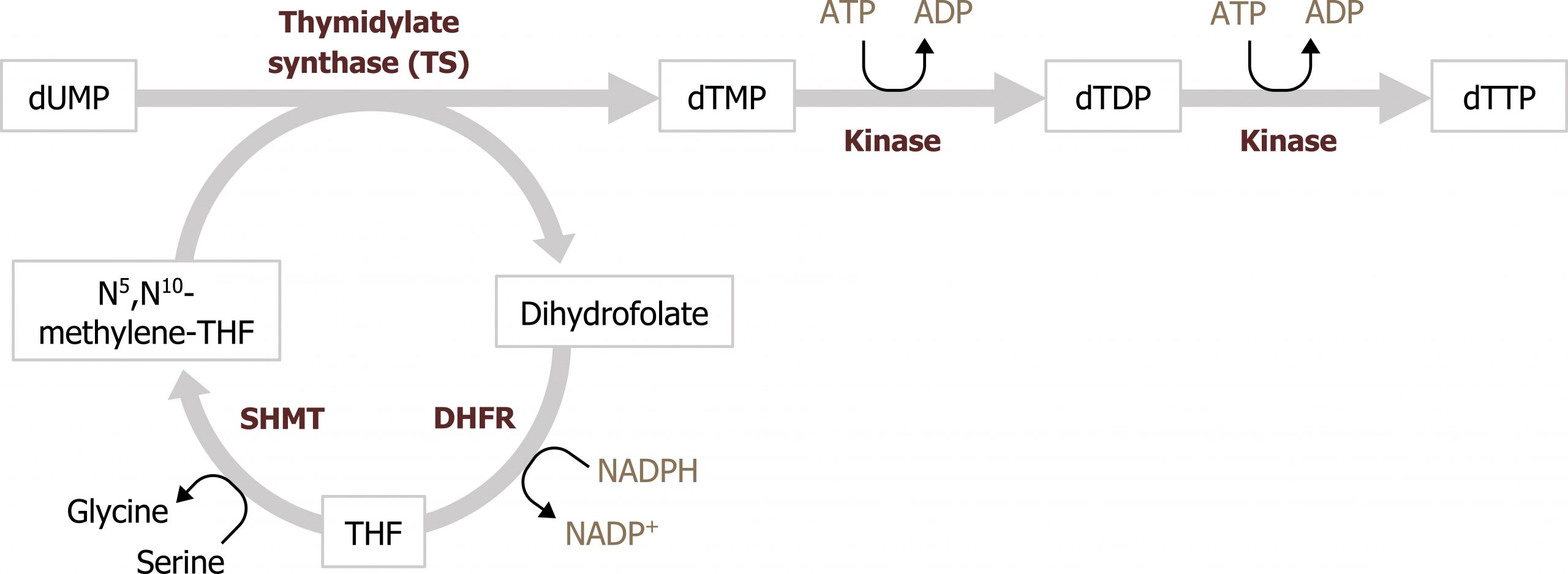
Defects in pyrimidine synthesis most commonly present as an increase in orotic acid in the urine. Deficiencies in the attachment of PRPP to orotate (or the decarboxylation of orotate monosphosphate) can result in the accumulation of orotic acid; similarly deficiencies of the urea cycle, which lead to an accumulation of carbamoyl phosphate, can increase flux through pyrimidine synthesis and cause an increase in orotic acid. Accumulation of orotic acid is used as a clinical indicator of pyrimidine deficiencies or deficiencies in the urea cycle.
Regulation of pyrimidine synthesis
The reaction catalyzed by CSPII is the regulatory step in the pathway and is activated by PRPP and ATP and inhibited by UTP.
Clinical importance of folate cycle inhibitors and synthesis of dTMP
Synthesis of dTMP for DNA synthesis is the rate-limiting step for the replication process, and therefore disruption of this conversion is very effective at reducing cellular proliferation. Inhibition of thymidylate synthase by 5-fluorouracil (5-FU) is a common anticancer treatment. 5-FU functions as a thymine analog and will irreversibly bind the enzyme. Similarly, methotrexate is an inhibitor of dihyrofolate reductase (DHFR), which is part of the folate cycle needed to reduce dihydrofolate to tetrahydrofolate. Inhibition of this process reduces substrate needed for the thymidylate synthase reaction and has a similar effect as inhibition of by 5-FU (figure 7.13).
Summary of pathway regulation
Metabolic pathway | Major regulatory enzyme | Allosteric effectors | Hormonal effects |
---|---|---|---|
Pyrimidine synthesis | CPSII | PRPP, ATP (+), UTP (-) | None |
Purine synthesis | PRPP synthetase | Pi (+), Purine bases (-) | Note: PRPP synthetase is required for both purine and pyrimidine synthesis |
Purine synthesis | GPAT | PRPP (+), IMP, AMP, GMP (-) | Note: PRPP synthetase is required for both purine and pyrimidine synthesis |
Table 7.2: Summary of pathway regulation.
7.2 References and resources
Text
Ferrier, D. R., ed. Lippincott Illustrated Reviews: Biochemistry, 7th ed. Philadelphia: Wolters Kluwer Health/Lippincott Williams & Wilkins, 2017, Chapter 13: Pentose Phosphate Pathway and NAPDH, Chapter 22: Nucleotide Metabolism.
Le, T., and V. Bhushan. First Aid for the USMLE Step 1, 29th ed. New York: McGraw Hill Education, 2018, 35–37, 79.
Lieberman, M., and A. Peet, eds. Marks’ Basic Medical Biochemistry: A Clinical Approach, 5th ed. Philadelphia: Wolters Kluwer Health/Lippincott Williams & Wilkins, 2018, Chapter 27: Pentose Phosphate Pathway, Chapter 39: Purine and Pyrimidine Synthesis.
Figures
Grey, Kindred, Figure 7.5 Overview of purine and pyrimidine bases. 2021. Chemical structure by Henry Jakubowski. https://archive.org/details/7.5_20210926. CC BY 4.0.
Grey, Kindred, Figure 7.6 Synthesis of PRPP and regulation of PRPP synthetase. 2021. https://archive.org/details/7.6_20210926. CC BY 4.0.
Grey, Kindred, Figure 7.7 Overview of purine synthesis. The reaction catalyzed by GPAT is the regulatory enzyme of the pathway. 2021. https://archive.org/details/7.7_20210926. CC BY 4.0.
Grey, Kindred, Figure 7.8 Purine synthesis and regulation of glutamine:phosphoribosylpyrophosphate amidotransferase. 2021. https://archive.org/details/7.8_20210926. CC BY 4.0.
Grey, Kindred, Figure 7.9 Breakdown of nucleotides. 2021. https://archive.org/details/7.9_20210926. CC BY 4.0.
Grey, Kindred, Figure 7.10 Nucleotide base salvage. Reaction catalyzed by HGPRT is clinically relevant as deficiencies can cause accumulation of uric acid. 2021. https://archive.org/details/7.10_20210926. CC BY 4.0.
Grey, Kindred, Figure 7.11 Nucleotide specific pathways for base salvage. 2021. https://archive.org/details/7.11_20210926. CC BY 4.0.
Grey, Kindred, Figure 7.12 Overview of pyrimidine synthesis. The reaction catalyzed by carbamoyl phosphate synthetase I is the regulatory enzyme of the pathway. 2021. https://archive.org/details/7.12_20210926. CC BY 4.0.
Grey, Kindred, Figure 7.13 Interaction of thymidylate synthesis with the folate cycle. SHMT: Serine hydroxymethyltransferase; DHFR: Dihydrofolate reductase. 2021. https://archive.org/details/7.13_20210926. CC BY 4.0.
Lieberman M, Peet A. Figure 7.4 Basic structure of nucleotides. Adapted under Fair Use from Marks’ Basic Medical Biochemistry. 5th Ed. pp 216. Figure 12.3 Nucleoside and nucleotide structures displayed with ribose as the sugar. 2017. Chemical structure by Henry Jakubowski.