5 Sensory Capabilities of Fish
Learning Objectives
- Recognize the adaptive significance of sensory capabilities of fish.
- Compare and contrast the sensory system of humans and fish.
- Relate the sensitivities of fish to the characteristics of the underwater world.
- Describe how sensory capabilities relate to the fish’s ability to communicate and orient.
- Express how the sensory abilities lead to responses to environmental stimuli.
- Apply concepts of fish sensory capabilities to predict effects of humans on fish.
3.1 Introduction
Fish may seem alien to us because they evolved in water and their senses are more adapted to an aquatic environment. Yet, like humans, fish depend on many senses for survival. Vision is a dominant sense in fish, and we humans can appreciate the capability for depth perception and color discrimination. But what happens when you attempt to see underwater? Your vision is very blurry underwater. Somehow fish solved the problem of seeing underwater. Sensory capabilities of fish are adapted to accommodate the special characteristics of the aquatic environment.
Imagine, if you will, a day in the life of a fish. Without eyelids, their eyes are open all the time. Daily cycles of light intensity are sensed by photoreceptors in the eye and pineal organ in the brain, which contains light-sensitive nerve endings. Vision is a dominant sense of fish that we humans can appreciate. Whether the fish finds a meal or becomes prey depends on many senses, such as the abilities to see, hear, smell, taste, and to detect water movement and electrical fields. Fish have a special sense that humans do not have: the ability to detect vibrations moving through water. Because sound vibrations move easily through water, fish do not need external ear openings, and yet they also have sensitive hearing.
Together, fish use these senses to inspect the world around them. Imagine an angler tossing a lure nearby. The fish will feel the vibrations caused by the waves moving from the lure. With wide-angle vision, the fish moves toward the lure to inspect it. With an acute sense of smell, it detects no signal that suggests it’s living. In some cases, the fish will grab a bait, taste it with sensitive taste buds, and reject it as nonfood. If captured, the fish has many sensory structures in the skin to detect touch and temperature changes.
But fish use sense for more than just finding food. Fish can rely on one or more sensory cues and different sensory mechanisms to gain information about their environment and guide their behavior. Senses are engaged whether the fish is moving toward a sound, away from a threat, or following a scent of food or pheromones. For example, young glass eels (Anguilla spp.) return to estuaries and detect currents using their magnetic compass to memorize magnetic direction of tidal flows (Cresci et al. 2019). As you learn more about the sensory capabilities of fish, you will be better able to understand their behavior.
3.2 Characteristics of the Water Shape Sensory Capabilities
Humans share some homologous organs and body parts with fish (Table 3.1). However, characteristics of water exert evolutionary pressures on fish to enhance their sensory capabilities in water. Water is dense, colorless, and odorless and can refract and reflect light waves in such a way that some colors are absorbed, particularly at deeper depths. Consequently, sound waves travel fast, scents are rapidly dissolved and detected in low concentrations, and vision is keen in fish that are active during the daytime. There is less oxygen dissolved in water than in the atmosphere. Therefore, gills are highly efficient at oxygen diffusion, and oxygen-sensing cells are sensitive at detecting changes in oxygen content of the water, sending signals to increase gill ventilation as oxygen declines. Similarly, terrestrial vertebrates have oxygen-sensing cells in the lungs to signal a change in breathing rate.
It’s not just the presence but also the location of sensory organs that reflects these evolutionary pressures (Figure 3.1). Fish smell with nares, far forward on the head, in front of the eyes, so that new scents are detected as the fish swims forward. Taste buds in fish are not restricted to the mouth but are distributed throughout parts of the body to allow the fish to taste its environment. Eyes are typically above the midline and on either side of the head, allowing fish a wide field of vision in front and along the sides and above—locations of typical predator threat. Water flow patterns are detected along the entire length of the body via sensory hair cells in the lateral line and other locations. In this way, the fish detects the flow field as it swims forward and detects disturbances in the flow field made by prey and predators. For example, when a fish detects the accelerating flows of suction or ram actions of predators, it will instinctively make a turn or C-shaped body bend and move in an opposite direction (Mirjany et al. 2011). The reaction occurs within 10 milliseconds.
Some fish have evolved a reduced or negative capacity for some senses to match their environment. Fish in muddy water habitats often have very small eyes because vision is less important. Some fish that live in dark caves have totally lost the sense of vision. Blind cavefish use the flow-sensing capabilities of their lateral line system rather than vision to avoid swimming into obstacles.
Human | Fish |
---|---|
Lungs | Gills |
Stomach | Stomach |
Liver | Liver |
Kidneys | Kidneys |
Ears | Lateral line, otoliths, and inner ear |
Skin | Scales and slime layer |
Nose | Nares |
Arms | Pectoral fins |
Legs | Pelvic fins |
Table 3.1: Homologous organs in humans and fish.
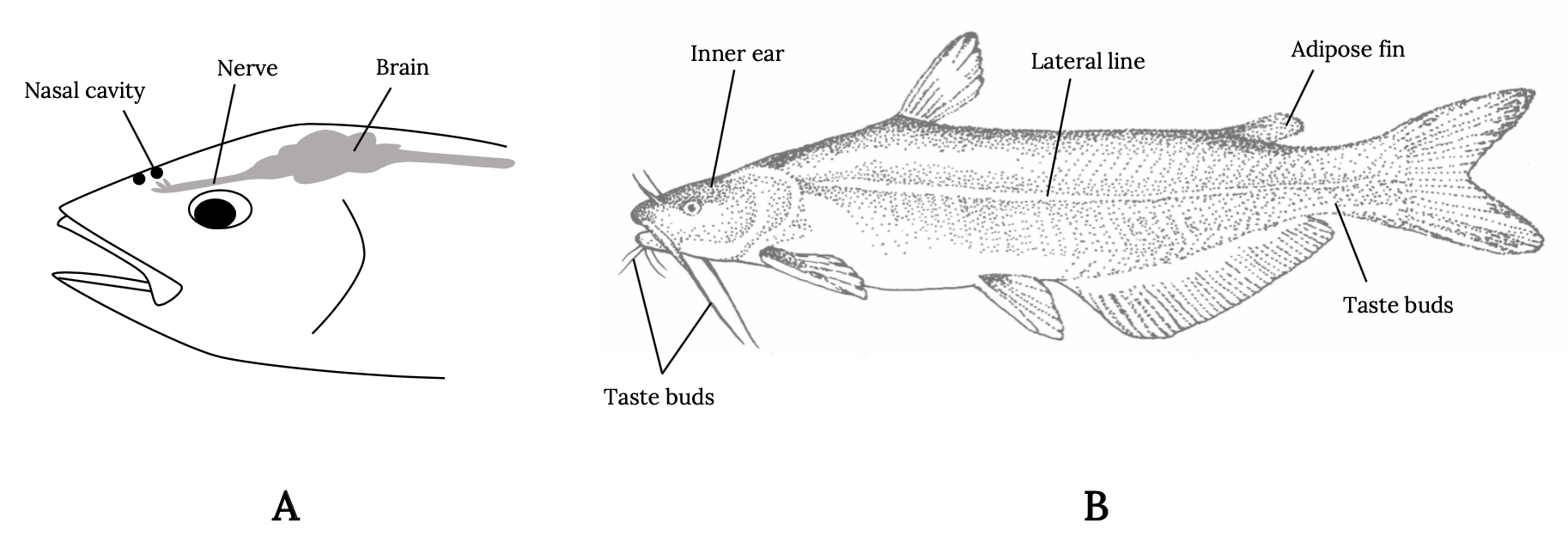
Question to ponder:
You are assigned a task at work to create the perfect marketable fish bait. Draw (with color) and describe the most ideal bait for either a catfish or a tuna. Describe how this will move through the water when fished and other features that would make it more marketable to anglers. Modify your design and description after you complete your reading of this chapter.
3.3 How We Study Sensory Ecology
Sensory ecology focuses on the study of animal sensory systems to understand how environmental information is perceived, how this information is processed, and how this affects interactions between the animal and its environment (Dangles et al. 2009). The stimulus-response model (Figure 3.2) describes the basic reactions from the stimulus, through receptors to the central nervous system and brain, which are then transmitted to neurons and organs that respond due to detection of the stimulus. A stimulus is any change in the environment (either external or internal) that is detected by a receptor. It may be a predator threat, an easy prey item, or a potential mate. Receptors transform environmental stimuli into electrical nerve impulses. These impulses are then transmitted via neurons to the central nervous system and brain where decision making occurs. When a response is selected (consciously or unconsciously), the signal is transmitted via neurons to effectors. Effectors are organs (either muscles or glands) that produce a response to a stimulus. A response is a change in the organism resulting from the detection of a stimulus.
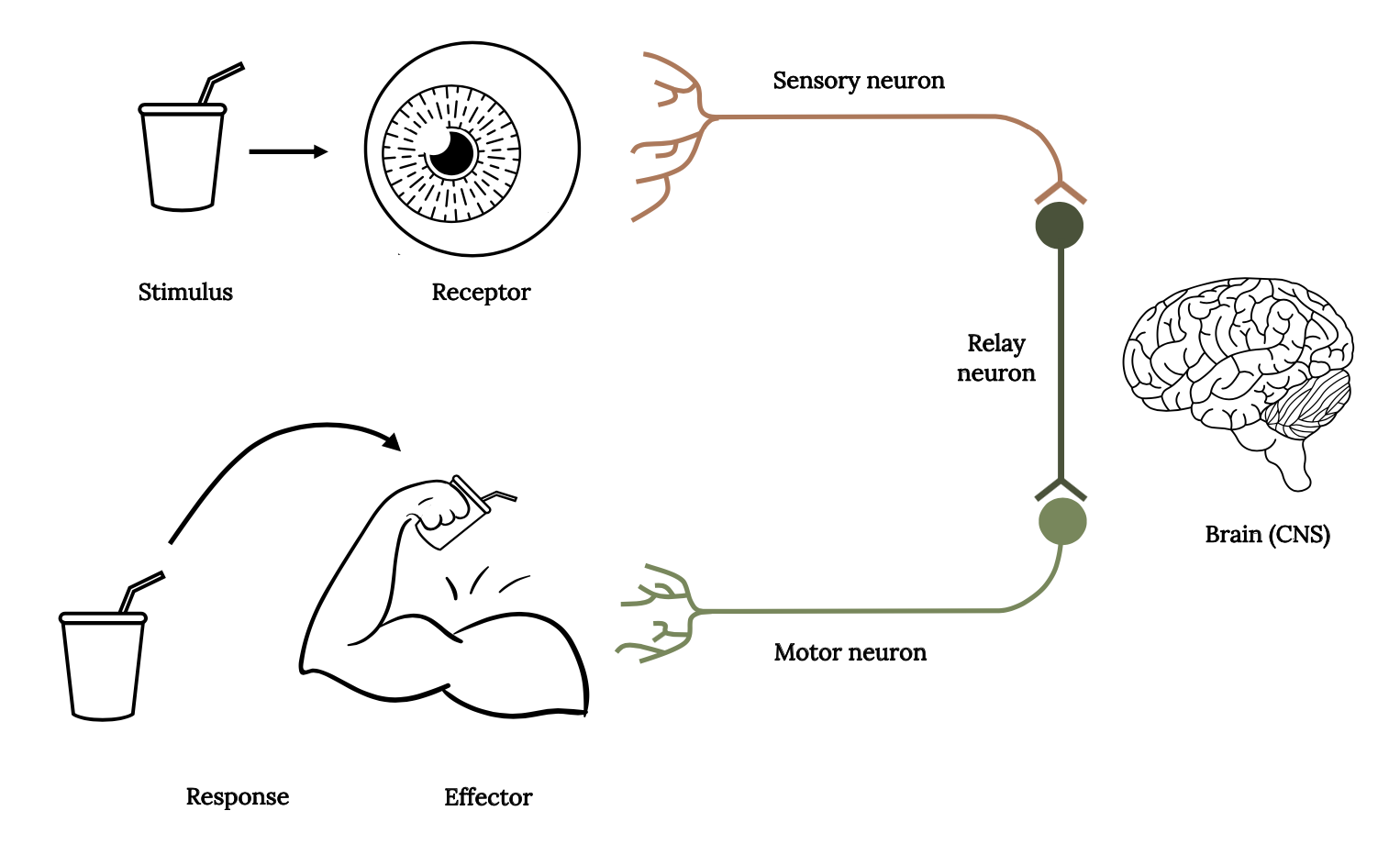
Three types of neurons are required to transmit information via the stimulus-response pathway: (1) sensory neurons transmit information from sensory receptors to the central nervous system (CNS); (2) relay neurons (interneurons) transmit information within the CNS as part of the decision-making process; and (3) motor neurons transmit information from the CNS to effectors (muscles or glands), to initiate a response.
The fascinating interplay between the different sensory abilities of the fish leads to their unique response to environmental stimuli that we observe. Consequently, biologists who study sensory ecology apply both behavioral and physiological approaches. The behavioral approach involves training or conditioning fish so that they respond to a stimulus. The fish is trained to do some tasks, such as move to one side of a tank, when it receives a stimulus such as a sound, a smell, or a visual cue. In this way, biologists can measure the reaction of fish to various stimuli.
The electrophysiological approach measures the responses to a stimulus by placing electrodes close to the nerve. The approach does not require any behavioral response by the fish; it only indicates that the stimulus was detected. The basic pathway for a nerve impulse is described by the stimulus-response model. The locations of sensory neurons of cutaneous taste buds of catfish were mapped in detail long ago (Herrick 1901), which allowed the first studies that exposed taste buds on the skin to various chemical stimuli and measured the responses in specific nerves via electrodes and amplifiers to display the electrical signal response (Hoagland 1933).
Catfish have a keen sense of taste and smell, and their taste buds are densely packed on the barbels, mouth, and skin. Barbels are particularly useful for catfish, as they literally “taste” the surrounding environment in the dark of night. As an example of the behavioral approach, a study of catfish in a large aquarium revealed that small catfish quickly responded to a small drop of pork juice and could locate the source with taste alone within 24 seconds (Bardach et al. 1967). The value of the behavioral approach is also revealed by a study that demonstrated the ability of sharks and rays to locate a flatfish buried in the sand by using their ability to detect weak electric fields generated by the hidden flatfish (Kalmijn 1971; King and Long 2020). Understanding the behavior of fish is of widespread interest, especially in the study of anthropocentric pollution that may obscure or interfere with detection of stimuli that fish use to make sense of their surroundings.
3.4 Distant Touch and Hearing
Humans hear sound when air molecules vibrate and move in a pattern called waves or sound waves. Fish have sensitive hearing that is adapted to the underwater environment, where sound waves move four times faster than in air because water particles are packed closer together. Because sound waves move faster in aquatic environments, the underwater world is filled with myriad sound sources that provide the fish with information from far greater distances than do other sensory stimuli. Fish use their hearing abilities to assess their surrounding soundscape and determine the availability of food, mates, or competitors, as well as the threat of predators (Putland et al. 2019). Fish may not hear the sounds of two anglers speaking in a boat because their sound waves are traveling through the air. However, fish will hear the propeller from an electric trolling motor from a good distance away.
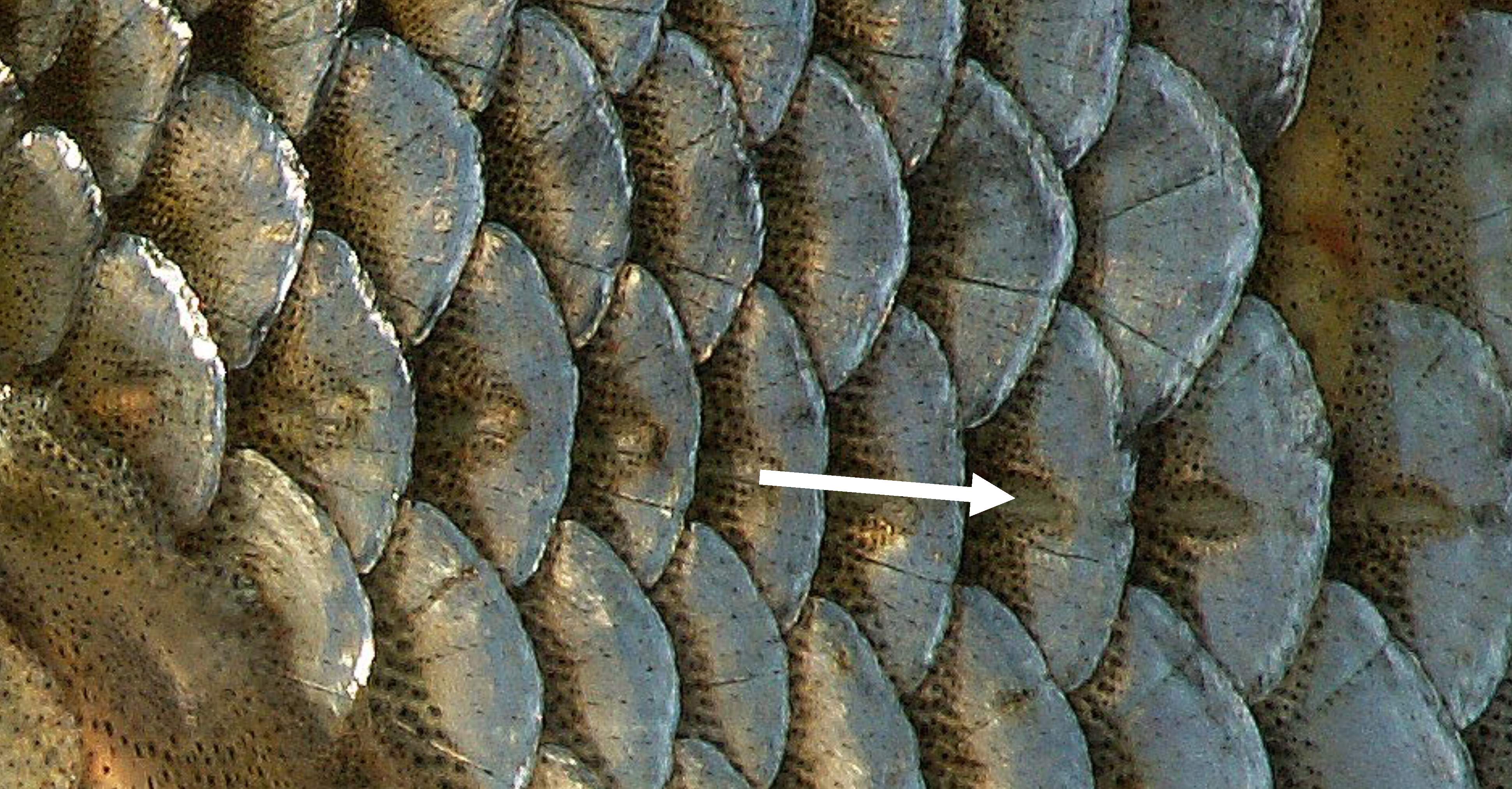
Sound perception is so critical to survival of fish that the hearing anatomy is fully developed within two days of hatching, when fish are just developing swimming and other sensory capabilities. Unlike humans, which have external ears, fish have two organs for hearing that are not obvious to the casual observer. Fish have an internal ear and an external lateral line system. The lateral line is an organ of microscopic pores primarily used to sense vibrations and pressure in the water (Figure 3.3; Montgomery et al. 2014). The pores are lined with neuromasts, which contain sensory hair cells (Figure 3.4). Each hair cell has bundles of cilia embedded in a gelatinous structure, called the cupula. Water movements deflect the cupula and cilia bundles, creating a change in membrane potential that is transmitted to the sensory neuron.
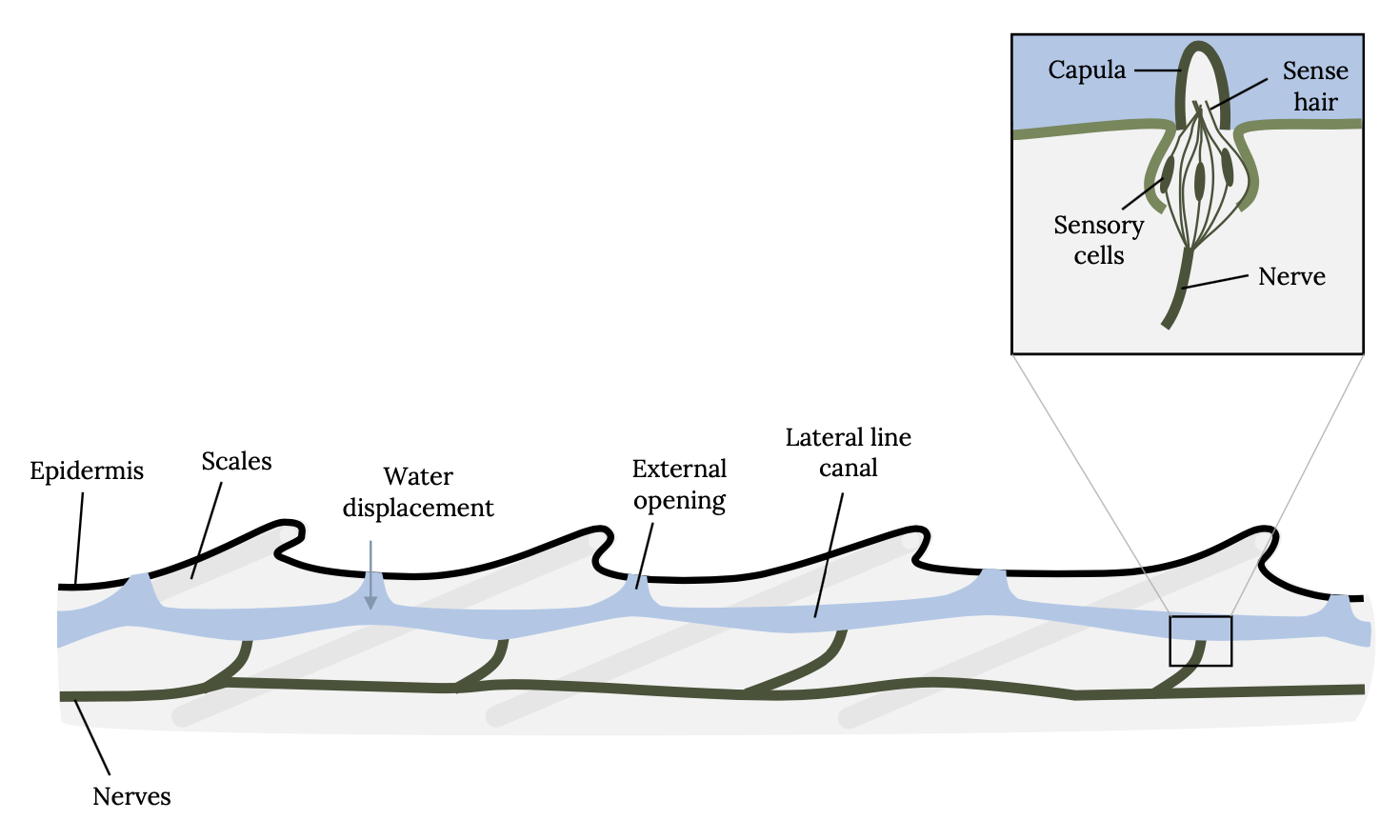
In addition to neuromasts found in the lateral line canal (see Figure 3.4), fish also have neuromasts in canals and on the surface of the skin in clusters on the head, trunk, and tail fin. The number and location of neuromasts influence the sensitivity. For example, the Goldfish has more superficial neuromasts and is more sensitive to water vibrations than the Rainbow Trout. Biologists have found that fish spend much of their time orienting their body, and the ability to sense local water movements is essential to the motion of the fish (Liao 2007; Coombs and Montgomery 2014). The small adipose fin, which is only present in some families of fish, detects water flow across the dorsal surface near the caudal region of the body and aids in swimming (Stewart and Hale 2013). Another unique specialization of neuromasts is the extended lateral line canal along the bottom jaw of the halfbeak fish, which allows it to detect, track, and intercept small but relatively fast-moving prey without using vision (Montgomery and Saunders 1985).
Fish utilize the lateral line to detect movements of prey, predators, currents, and objects in the water. If there is any difference between the relative movements of the body of the fish and the movements of the surrounding water, it will be sensed by the lateral line (Mogdans 2019). In this way, the fish knows if it is swimming in highly turbulent or still waters. The lateral line is also very sensitive to water vibrations from great distances underwater, so this sixth sense is sometimes called the far-field hearing (Figure 3.5).
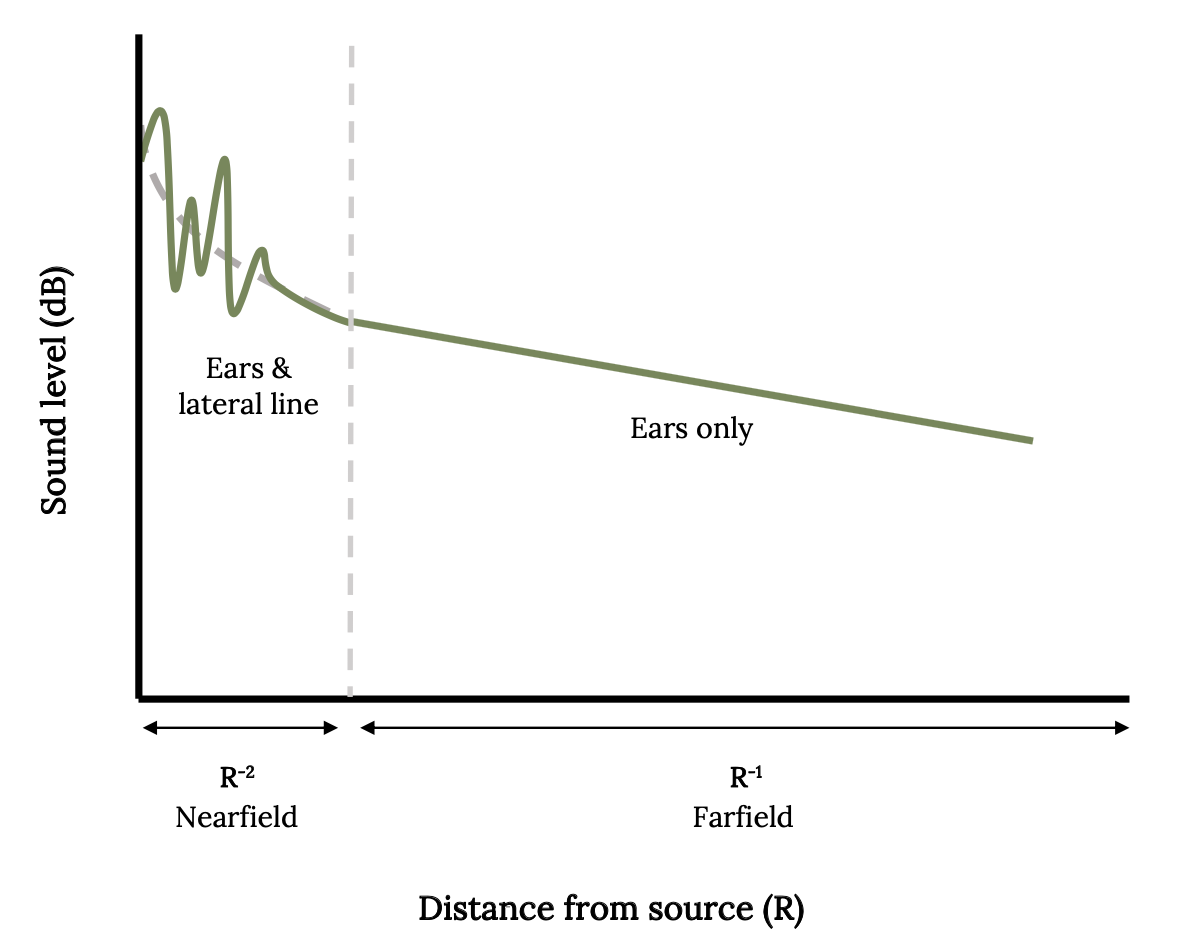
The inner ear of bony fish consists of semicircular canals connected to organs with otoliths, or ear stones (Figure 3.6). It is similar to the cochlea in humans and other vertebrates. When sound waves go through a fish, the denser ear stone moves more slowly and the sensory hair cells and cilia are deflected, thereby sending signals to the brain. Some deflections are interpreted as sounds, and some signal acceleration of the fish (Tavolga et al. 1981; Popper and Schilt 2008; Popper et al. 2019). Swim bladders in bony fish vibrate as well, and direct connections to the inner ear enhance the hearing sensitivity in certain fish, such as Goldfish.
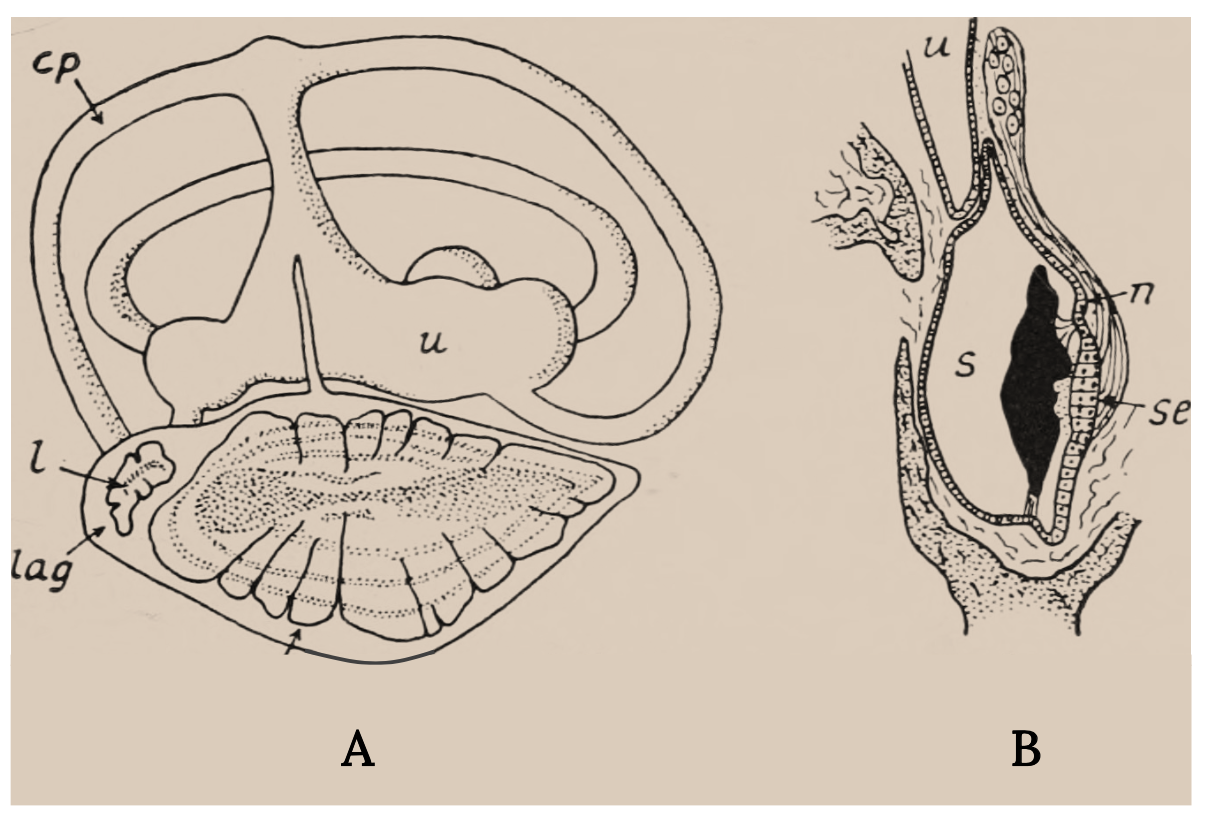
Far-field and near-field hearing are adaptations for increased survival, feeding success, and breeding in fish. During the early development of many fish that occupy coral reefs, the planktonic larvae drift in the currents. Drifting larval fish use sounds produced by different underwater habitats to orient and locate suitable habitats to settle into. Furthermore, some fish use sound to discriminate between habitats when moving from sheltering to feeding habitats at night. Herring and shad have elongated gas ducts that extend from the swim bladder to the skull, which enhances hearing to ultrasound up to 100,000 Hz, overlapping the range of echolocation sounds of dolphins and porpoises. When American Shad hear ultrasonic clicks like those of dolphins, the fish either swim in the opposite direction of the sound source or move chaotically, making it harder for the dolphin to detect and capture the fish (Mann et al. 1997). Herring also escape approaching predators by detecting changes in water flow that the predator causes. In many sound-producing fish, the males produce sounds to attract a receptive female in initial courtship interactions. For example, females tend to choose males based on calling rate or effort, which is linked to body fat reserves and gonad size. Consequently, these and many other examples demonstrate how fish survival and breeding success are enhanced by specialized hearing.
3.5 Vision
Every experienced angler is aware of the keen vision of fish and uses this knowledge to catch more fish. The eyes of most fish are placed laterally on the head and tilted forward and upward. Vision is therefore nearly absent downward and to the rear, providing wide-angle monocular vision and a narrow zone of binocular vision. Consequently, for most sport fish, the angler’s best line of attack is directly behind in the blind zone (Figure 3.7A). The angler also knows that light waves are reflected, refracted, or absorbed, depending on the angle. Therefore, fish have a cone-shaped range of vision that is approximately two times the depth of the fish. Outside this cone, the fish sees nothing and the angler’s approach is hidden. The water surface around the window is either black or a mirror, depending on the angle at which light rays are reflected (Figure 3.7B).
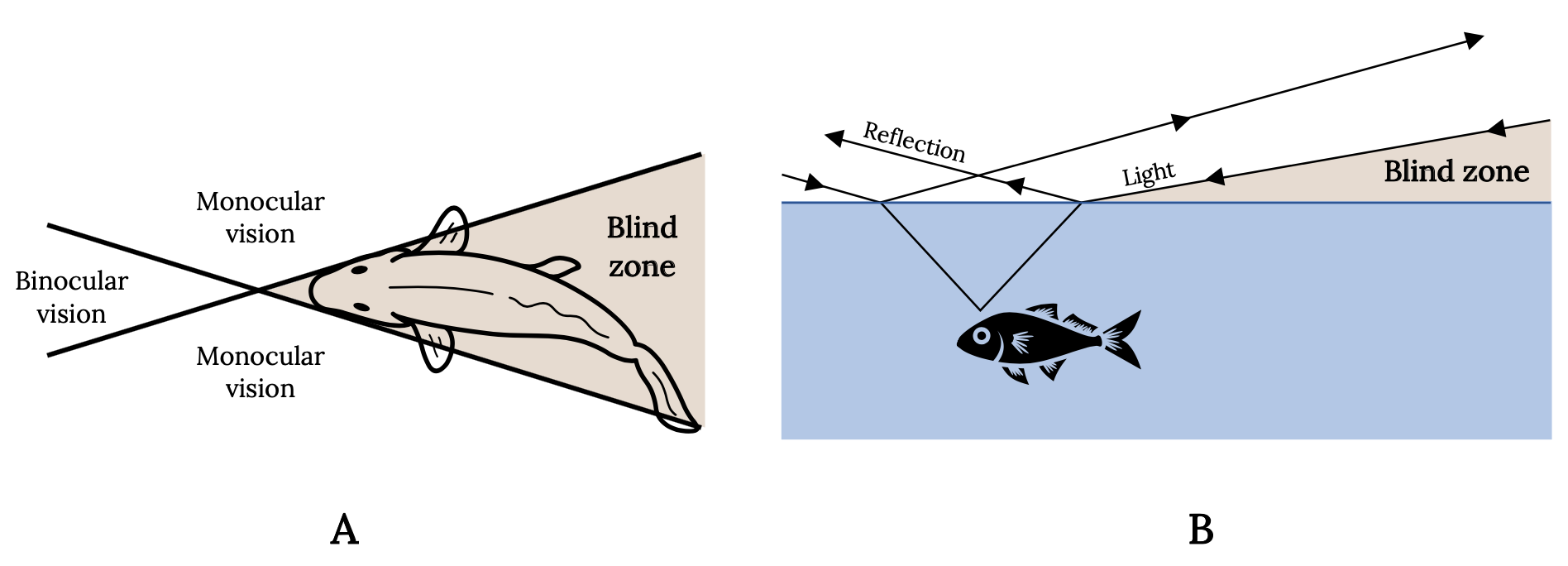
Tips for stalking fish based on fish vision (Mayer 2019):
- Bottom brim of your hat should be a dark color.
- Wear dark or camouflage clothing and avoid wearing shiny objects.
- Wear polarized sunglasses that cover the sides of your eyes thoroughly.
- Block unwanted reflected rays by placing your arm beneath your chin.
- Keep the sun at your back and watch your shadow.
- Approach from downstream and keep a low profile.
- Place your fly upstream and within the binocular zone (a 30–36° angle) of a position-holding trout.
- Remember that the fish is holding position deeper and closer than it looks.
The eye of a fish has a similar anatomy to humans and consists of a cornea, iris, lens, sclera, choroid, and retina and is filled with a gel (or vitreous humor) between the lens and the retina (Figure 3.8). However, a fish’s eye operates differently than that of a human to accommodate underwater sight. The lens of a fish’s eye is purely spherical, unlike ours, and it has a refractive index (light-bending ability) of 1.65, which is higher than that of any other group of vertebrates. The lens focuses light waves on the retina, which influences the sensitivity of fish vision (Li and Maaswinkel 2006). Furthermore, the lens is fixed in its shape, meaning its shape cannot be adjusted to facilitate focusing on nearer or more distant objects. Therefore, unlike humans, most fish adjust focus by moving the lens closer or further from the retina. Bony fish do so by contracting a muscle.
Also like humans, the retina of the fish’s eye is made up of photosensitive rods and cones. The rods detect only the presence or absence of light, and the cones detect color (Douglas and Djamgoz 1990). Most bony fish can detect color (Marshall et al. 2017). Most sharks, however, have only rods, and therefore they distinguish contrast, not color. In most bony fish, rods for low-light vision are much more common than cones, which are better for bright-light vision. As a general rule, the deeper a fish lives the fewer cones it has.
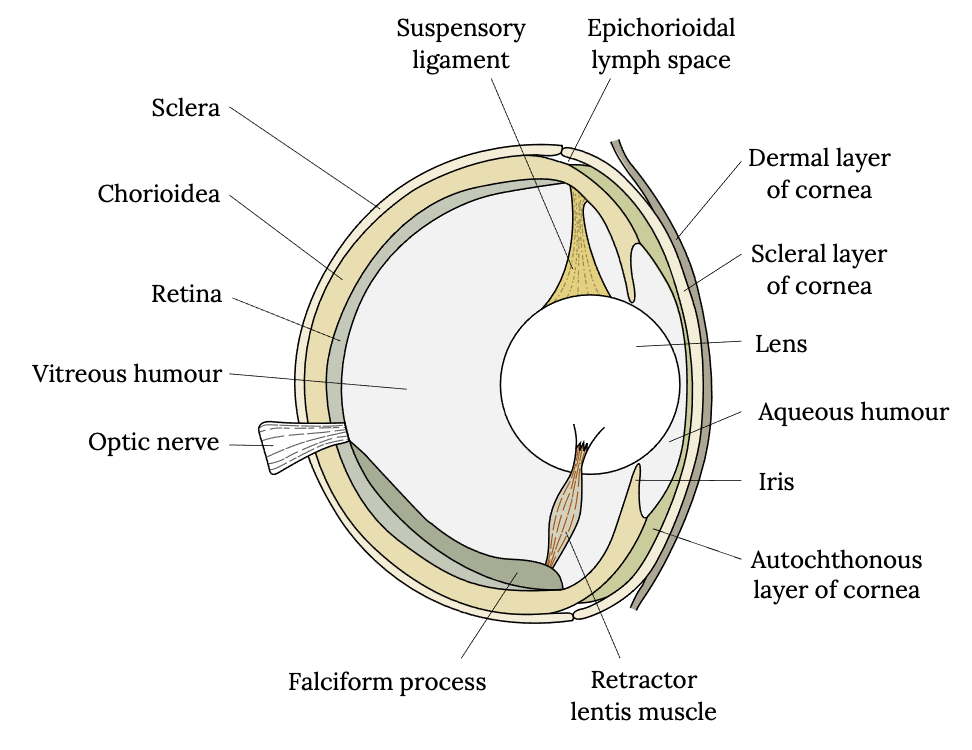
Color vision in fish is a hotly debated topic for some anglers. This is because fish do not see colors as humans do, and color sensing is highly variable among different fish. Walleye, for example, are adapted to low-light conditions, and their eyes have more rods than cones, whereas Rainbow Trout color vision is more like human color vision. Furthermore, different color wavelengths travel through water differently. Longer wavelengths disappear more quickly than shorter waves. Therefore, the depth of fish will influence light penetration and color availability. The vivid reds and yellows on your fishing lure will lose that brightness as long wavelengths are absorbed in deeper water. In turbid water, light waves disappear even quicker. Commercial gill-net fishers dye nets blue or green so they blend into the background color in very deep water. The subject of color selection for trout angling is fully explored in many books, such as The New Scientific Angling: Trout and Ultraviolet Vision; What Fish See: Understanding Optics and Color Shifts for Designing Lures and Flies; and Trout Sense: A Fly Fisher’s Guide to What Trout See, Hear, & Smell. For the angler, it is often more about the contrast that the lure provides than the colors.
Two visual capabilities of some bony fish include polarization and ultraviolet (UV) vision. It is at dusk and dawn when the maximum amount of polarized light is refracted in water, and much of it is in the UV wavelengths. Humans perceive polarized light as glare. However, certain fish can discriminate polarized light. Damselfish, clownfish, trout, minnows, and anchovy may use this ability to enhance detection of small prey within the fish’s field of vision (Kamermans and Hawryshyn 2011). Many shallow-dwelling fish are capable of detecting UV radiation. Because humans cannot see UV light, the significance of this capability was initially a mystery. Scientists hypothesized that the ability of some fish to see UV reflections could represent a secret communication channel, hidden from predators. In coral reef fish, visual communication is a key mechanism for recognizing members of the same species. Experiments with damselfish demonstrated that they use their UV capabilities to discriminate between UV facial patterns of closely related species and their own (Siebek et al. 2010). These same facial patterns are invisible to humans and other fish but provide a hidden communication signal in damselfish.
Question to ponder:
Consider your favorite fishing target and their preferred habitat. How do you expect that the ability to hear (near and far) and vision influence your preferred choice of fishing lure or bait?
If you do not have a favorite fishing target, consider the Walleye and review this website.
3.6 Taste and Smell
Fish have an especially sensitive system for taste and smell, which have been well studied (Zielinski and Hara 2006; Kasumyan 2019). Smell occurs in the sensory folds with the nares on the head of a fish. However, fish are unique among vertebrates because they have taste buds that occur in many locations, which means a fish can literally taste its environment or food without putting it in its mouth. Taste buds can be seen on fish with a magnifying lens and appear as small pores with sensory cells connected to nerves (Figure 3.9). Fish have the highest number of taste buds recorded for vertebrate animals, and the amount of neural tissue devoted to sensing taste approaches 20% of the entire brain mass in some fish (Kotrschal and Palzenberger 1992). Fish are unique among vertebrates because they have external taste buds on their body in addition to taste buds on the lips and mouth. As early as 1827, taste buds of Common Carp were first described, and subsequently distribution of taste buds was studied in many other freshwater and marine fish. The location of taste buds on the body, barbels, or fin tips means that the fish can taste its environment as it moves and adapt or orient to potential food (Bardach and Atema 1971; Burton and Burton 2017). Studies frequently noted that the fish excelled humans in tasting tested substances and surprisingly showed a strong response to human saliva (Konishi and Zotterman 1961)—so there’s no evidence to support the idea that spitting on your bait brings good luck.
In some bony fish, taste and smell are dominant sensory modalities. In fact, some substances are both tasted and smelled. Taste sensors detect the presence and location of distant food sources. However, taste and smell are not just for feeding: they can also play a role in the protection of the young and in courtship.
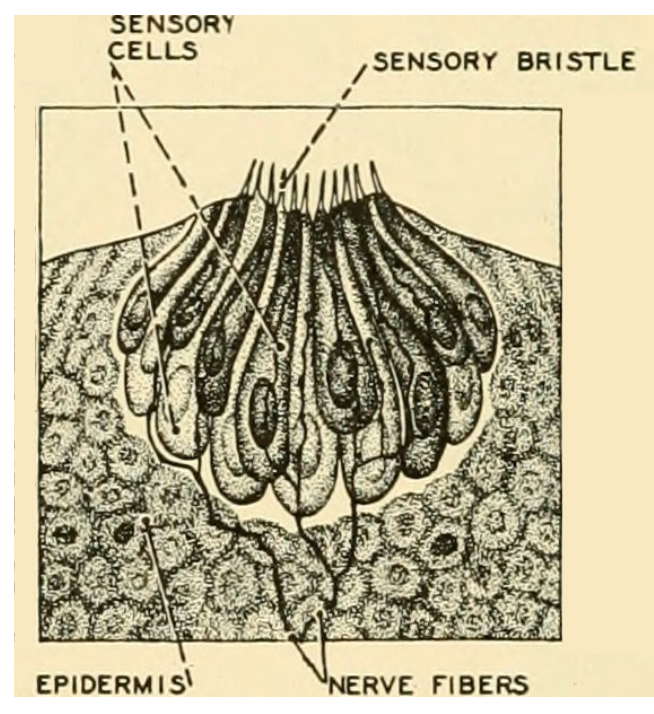
The key drivers for feeding are hunger and satiety. What is chosen to eat, however, is not determined solely by physiological or nutritional needs but by other factors such as the sensory properties of food. An encounter with food odor evokes feeding agitation and searching activity in fish and in most cases precedes grasping of the detected food item. The odor of familiar or habitual food makes fish grasp and test many previously indifferent dietary items, even those that in size, shape, or coloration only distantly remind the fish of real food.
The fact that odors attract certain fish has been used by recreational and commercial fishers for a long time. Worms are often kept in damp coffee grounds because the coffee smell attracts fish. Many baits and smelly fish are used in hoop nets and traps to attract catfish, lobsters, and crabs. Trout anglers have used garlic-scented marshmallows and corn for years because they work. Numerous scents are infused in formulated baits, such as Powerbait® and Gulp®. Many oils, such as menhaden milk, herring oil, shrimp oil, and squid oil, are used as fish attractants.
Serious catfish anglers have their favorite, secret recipe for stink baits made from liver, shad guts, old cheese, peanut butter, garlic, and many other aromatic foods.
The taste buds within the mouth allow fish to demonstrate strong and stable preferences for some foods. Fish quickly spit out a nonfood or nonpreferred food item. For example, common amino acids (L-alanine, L-cysteine, L-aspartic acid, glycine), sugars, and citric acid are preferred substances, and formulated diets for captive fish often use this information to formulate palatable artificial feed (Konishi and Zotterman 1961).
In addition to finding food, bullhead catfish use their sense of smell not only to identify but also to remember individuals in a group. This sense helps us to explain the development of dominance hierarchies in the bullheads. In dominance hierarchies, catfish will know one another; there will be one dominant catfish and others known as subordinates. The behaviors and recognition depend on the chemical cues, because even blinded individuals are capable of social recognition (Todd 1971).
Question to ponder:
Why might it be a good thing that fish have a keen sense of taste and do not consume everything that enters their mouth?
3.7 Electrosensory and Magnetosensory Capabilities
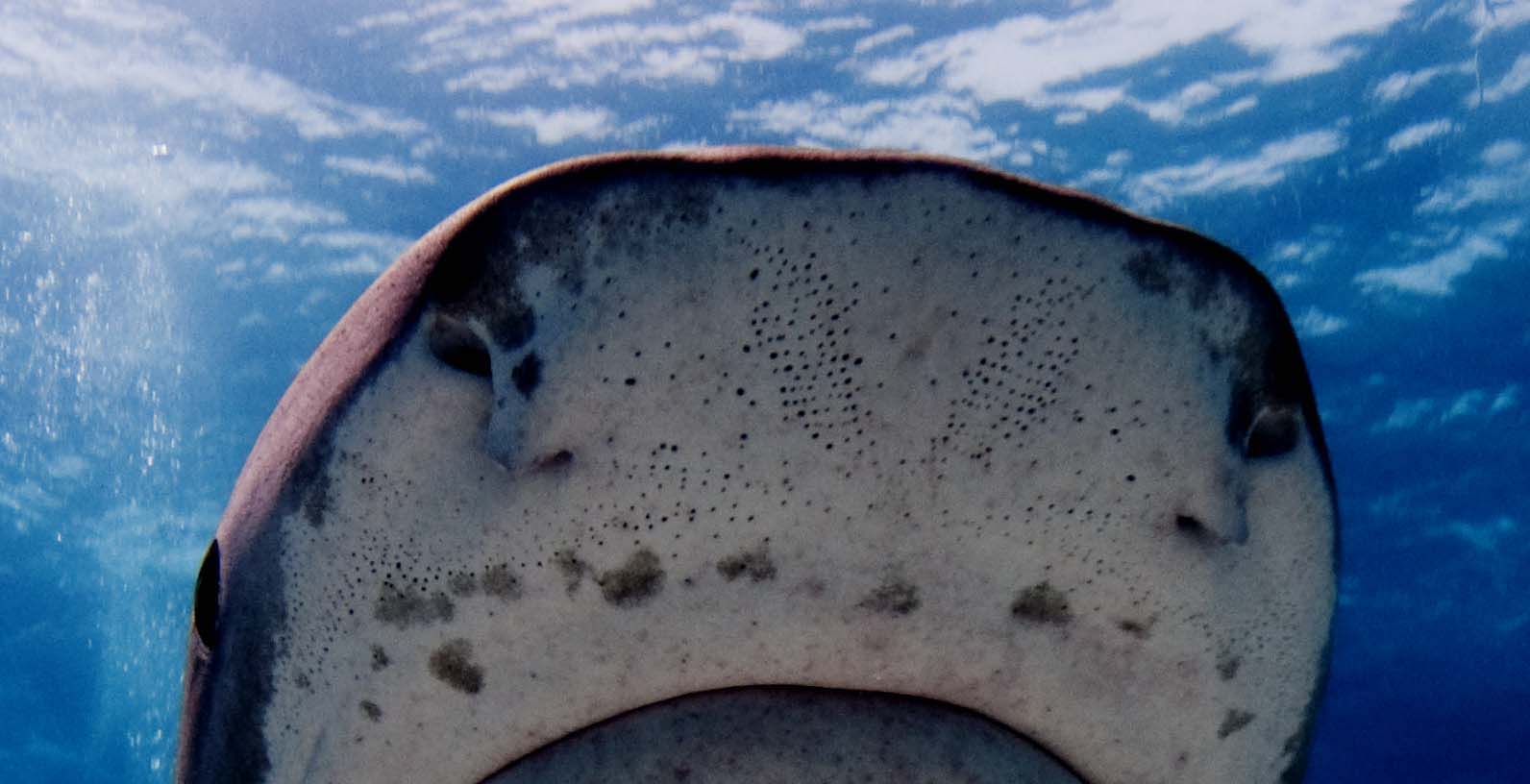
Some fish can receive signals from weak sensitive fields. Imagine swimming along the ocean bottom and sensing a live, hidden fish that you cannot see nor smell. Sharks are more sensitive to electric fields than any other animal, responding to charges from weak electrical potentials generated by muscle contractions of marine fish (Newton et al. 2019). Sharks, skates, and rays have hundreds or more small pores, known as ampullae of Lorenzini, that detect electric fields in the water (Figure 3.10). The pores are filled with a conductive gel that allows the potential to be transmitted to the nerve. This stimulus may be interpreted as a prey nearby, and the shark can orient toward the prey that is generating electric fields. Pores are primarily located around the mouth and body in sharks, skates, and rays to allow the fish to orient toward the prey (Collin et al. 2016). A secondary function of electroreception is detection of predators in less mobile juveniles.
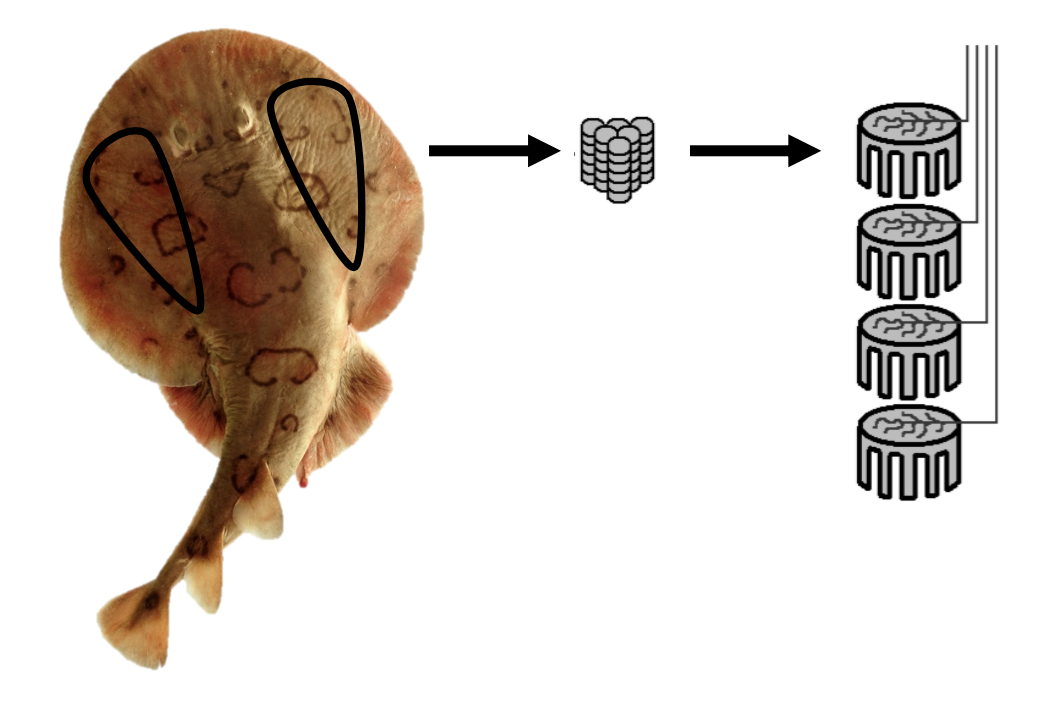
Certain electrosensitive fish also have an electric organ that generates a very weak electric field (Figure 3.11). The electric field generated helps the fish to navigate, communicate, incapacitate prey, and defend the fish from predators. Common examples include the electric ray, African elephantnose fish, and South American knifefish. Elephantnose fish can switch between relying on visual and electric sense, just as humans switch between sight and touch sensors. Processing electrical senses requires a very large brain and, therefore, electric fish use more oxygen for brain functions compared with humans. Interestingly, the brain size in Peters’s Elephantnose Fish is 3% of body mass, which is higher than the brain/body mass ratio of 2% in humans (Nilsson 1996).
Because of their electrosensitivity, sharks avoid certain rare-earth elements, such as lanthanide, which have magnetic properties. Experiments are ongoing to test whether certain metals or strong magnets can induce sufficient avoidance that they may be used for reducing bycatch in certain fishing gears (Richards et al. 2018).
Many fish possess the ability to detect and respond to the direction and intensity of magnetic fields (Formicki et al. 2019). Discovered relatively recently, the magnetic sense helps to explain the predictability of long-distance migration patterns observed in eels, sharks, tuna, and salmon. Salmon respond to the magnetic field with magnetoreceptor cells located in the nose of the fish.
Question to ponder:
How are the senses different from a shark (an open-water predator) and a flounder (benthic predator)?
3.8 Nociception
Nociception is the detection of harmful or unpleasant stimuli. When exposed to any harmful substance, fish make a reflex reaction and quickly withdraw. Stimuli that could cause tissue damage include high mechanical pressure, extremes of temperature, acids, venoms, and prostaglandins. While nociception is underexplored in fish, the free nerve endings (nociceptors) exist in the skin of Rainbow Trout, Zebrafish, Common Carp, and Goldfish and act as an alarm system to alert the fish to potential harm (Sneddon 2007). In the next chapter, the issues of pain and welfare are introduced.
3.9 Sensory Orientations
Variation in sensory capabilities is extremely high among groups of fish. This variation reflects the fact dominant habitats and habits are exhibited by different fish along with their evolutionary history. The jawless hagfish and lampreys are the oldest lineages of fish and have well-developed olfactory bulbs and a prominent brain stem, yet other senses (including sight) as measured by the size of brain parts are less developed. Cartilaginous fish (sharks, skates, and rays) have a highly developed sense of odors, as well as electric fields. In the bony fish, those that colonized and occupy a bottom-dwelling or benthic lifestyle, often have enhanced senses of taste, smell, and touch, which are more important than vision. Fish of clear water lakes and streams have excellent vision (Kotrschal and Palzenberger 1992; Kotrschal et al. 1998). Fish adapted to large, turbid rivers and estuaries have enhanced senses of taste and smell. Open-water fish rely more heavily on the lateral line sense. The cichlids and butterfly fish rely on integrating multiple sensory capabilities to allow life in complex spatial and social relationships. Therefore, we should never conclude that all types of fish have the same sensory specializations. Rather, sensory capabilities reflect the dominant habitats and way of life.
3.10 Sensory Disruptions and Human Presence
When we think of pollution to aquatic environments, we often picture images of trash and dirty water. However, human activities also add sensory pollutants that alter aquatic environments in ways that decrease the ability of fish to sense their underwater world. Imagine a world in which one or more of your senses was eliminated or greatly impaired by interference of some kind. In coastal embayments that surround ports and harbors, the ambient sound level is estimated to double in intensity every decade (Merchant et al. 2012). Further, pollution, toxicants, anthropogenic noise, and dams are human modifications that may influence sensory functions, depending on degree and type of change. Fish, in habitats greatly altered by humans, experience what biologists are calling sensory smog from many artificial stimuli (Preston 2019). Motorboats, pile driving, seismic airguns, and other activities produce sounds that may drown out other sounds. Ocean acidification influences the sense of smell in sea bass, decreasing their chances of detecting food or predators (Porteus et al. 2018). Ocean acidification and reductions in dissolved oxygen alters otoliths, which may affect sensory development (Simpson et al. 2011; Hamilton et al. 2019). Water pollution decreases underwater visibility and interferes with feeding and communication in fish (Fisher et al. 2006). Toxicants may influence the sensitive organs involved in taste and smell. Mitigation measures may protect animals from impacts of human activities. For example, activities that produce sound may be undertaken in ways that will reduce not just the levels and characteristics of the sound but also their effects on aquatic animals (Popper et al. 2020). Artificial light levels at night alter the biorhythms of fish, raising concerns that fish in urban waters may have impaired sleep (Kupprate et al. 2020). The future global changes will likely influence sensory behavior in fish, and many gaps in our understanding remain (Draper and Weissburg 2019; Dominoni et al. 2020).
Profile in Fish Conservation: Andrij Z. Horodysky, PhD
Key Takeaways
- Understanding and appreciating the diversity of fish requires that we know some basics of sensory capabilities and the ability to learn.
- The stimulus-response model for understanding sensory systems in fish is the same model used for all vertebrate organisms.
- Fish have excellent systems for hearing as well as a lateral line for detection of far-field water movements.
- Senses of smell and taste are well developed in fish, and there are many applications of that information in formulating artificial feeds and baits for fishing.
- Fish that live in shallow, clear waters often see well in color, while other fish may see contrasts in low-light conditions.
- Electrosensory perception evolved in a number of unrelated groups of fish and permits enhanced prey detection and capture.
- Some fish can generate an electric field, which is used for communication, defense, and foraging.
- Fish senses differ among fish based on their preferred environment.
- Human activities may interfere with some sensory systems of fish, and many gaps in our understanding limit our ability to predict the influence of global changes.
This chapter was reviewed by Andrij Z. Horodysky and Brendan J. Runde, PhD https://www.brendanrunde.com
URLs
Walleye: https://ladiesofangling.com/nicole-stones-walleye-fishing-setup/
Horodysky: https://home.hamptonu.edu/science/
Long Descriptions
Figure 3.1: Left: Simple drawing of fish showing location of nasal cavity in front of eye, a nerve above eye, and brain behind eye at back of head. Right: A more complex drawing of a fish shows location of taste buds in protruding appendages from chin, inner ear above and behind eye, lateral line at the center of main body, adipose fin before back fin, and taste buds on underside close to back fin. Jump back to Figure 3.1.
Figure 3.2: Drinking cup is a stimulus, arrow points to eyeball as receptor, nerves attached to a line is sensory neuron leading to a circle and line representing relay neuron, (brain to the right of line), leads to motor neuron, with nerves leading to a muscled arm lifting the drinking cup as effector, the drinking cup being brought up by the arm is the response. Jump back to Figure 3.2.
Figure 3.4: Drawing of close-up lateral line of fish, including epidermis, nerves, scales, water displacement, external opening, and lateral line canal. Movements of water in canal cause capula to move, to stimulate sensory hair cells. Jump back to Figure 3.4.
Figure 3.5: Line graph depicts the sound levels detected by fish by inner ear and lateral line when sound source is near, which are higher than sound levels detected by inner ear alone when sound source is distant. Jump back to Figure 3.5.
Figure 3.6: Two line drawings, one depicting inner ear and the other depicts close up of sacculus with labels for posterior semicircular canal, statolith of lagena, lagena, nerve;sacculus, sensory epithelium and utriculus. Jump back to Figure 3.6.
Figure 3.7: Two diagrams that show top and side views of how light waves are refracted and resulting field of vision and blind spots for fish. Blind spot is behind the fish. Jump back to Figure 3.7.
Figure 3.8: Drawing of vertical cross section of fish eye, including dermal layer of cornea, scleral layer of cornea, lens, aqueous humour, iris, autochthonous layer of cornea, retractor lentis muscle, falciform process, optic nerve, vitreous humour, retina, choroidea, sclera suspensory ligament, and epichorioidal lymph space. Jump back to Figure 3.8.
Figure 3.11: Photograph of an electric ray with two upside down triangles drawn on either side of the eyes, an arrow leads to a drawing of stacked coin shapes to represent electrocytes, and an arrow to four drawings of stacked electric organs. Jump back to Figure 3.11.
Figure References
Figure 3.1: Locations of sensory structures on the body of a fish. (A) Nares, eye, pineal, and brain locations. (B) Inner ear, lateral line, adipose fin, and taste bud locations. Kindred Grey. 2022. Adapted under fair use from “Ocean Fish Are under Threat if We Don’t Curb Carbon Dioxide Emissions,” by Cosima Porteus, 2018 (https://theconversation.com/ocean-fish-are-under-threat-if-we-dont-curb-carbon-dioxide-emissions-107312). Includes Blue Catfish by Louisiana Sea Grant College Program Louisiana State University, 2007 (https://flic.kr/p/2A7sP1).
Figure 3.2: Diagram of the connections in the stimulus-response model in fish, which displays a stimulus, odor receptor (nares), sensory neuron, relay neuron, motor neuron, brain, effector, and response. Kindred Grey. 2022. CC BY 4.0. Includes drinking glass by sumhi_icon, 2017 (Noun Project license, https://thenounproject.com/icon/drinking-glass-1274096/), bicep muscle by Vectors Point, 2020 (Noun Project license, https://thenounproject.com/icon/bicep-muscle-3149162/), eyeball by ME, 2017 (Noun Project license, https://thenounproject.com/icon/eyeball-931632/), brain by Mahmure Alp, 2019 (Noun Project license, https://thenounproject.com/icon/brain-2300842/).
Figure 3.3: Scales along the lateral line (see arrow) of the Roach Rutilus rutilus. Piet Spaans. 2006. CC BY-SA 2.5. https://commons.wikimedia.org/wiki/File:RutilusRutilusScalesLateralLine.JPG.
Figure 3.4: Schematic of the lateral line system of fish. Movements of water in the lateral line canal cause the cupula to move, thereby stimulating sensory hair cells connected to nerves. Kindred Grey. 2022. CC BY SA 3.0. Adapted from LateralLine Organ by Thomas.haslwanter, 2012 (CC BY-SA 3.0, https://commons.wikimedia.org/wiki/File:LateralLine_Organ.jpg).
Figure 3.5: Sound level in decibels plotted as a function of distance from the source. Kindred Grey. 2022. Adapted under fair use from “The Potential Overlapping Roles of the Ear and Lateral Line in Driving ‘Acoustic’ Responses,” by Dennis M. Higgs and Craig A. Radford, 2016 (https://doi.org/10.1007/978-3-319-21059-9_12).
Figure 3.6: (A) Labyrinth of a flying fish (Exocoetus). (B) Section through the sacculus of the trout. Key structures. Nicol, J. A. Colin. c. 1960s. Public domain. https://flic.kr/p/wLFLig.
Figure 3.7: Diagram shows the refraction of light at the interface of air and water and the cone-shaped range of vision in the fish. (A) Top view. (B) Side view. Kindred Grey. 2022. Adapted under fair use from “Some Important and Interesting Aspects about Yellowfish” (https://www.fishingowl.co.za/flyfishyel2.html) and “The Science of Stalking Fish,” by Alan Bulmer, 2017 (https://activeanglingnz.com/2017/02/01/the-science-of-stalking-fish/). Includes Goldfish top view by Oleksandr Panasovskyi, 2020 (Noun Project license, https://thenounproject.com/icon/goldfish-top-view-3635952/) and “Fish,” by Kangrif, 2017 (Noun Project license, https://thenounproject.com/icon/fish-1186818/).
Figure 3.8: Diagrammatic vertical section through the eye of a teleost fish, after Walls (1942). Kindred Grey. 2022. CC BY-SA 4.0. Adapted from “Bony Fish Eye Multilang,” by Gretarsson, 2019 (CC BY-SA 4.0, https://commons.wikimedia.org/wiki/File:Bony_fish_eye_multilang.svg).
Figure 3.9: Diagram of the taste buds in fish. Herbert Vincent and Herbert Wilbur. 1939. Public domain. https://flic.kr/p/wsuopv.
Figure 3.10: Pores with ampullae of Lorenzini in snout of Tiger Shark. Albert kok. 2009. CC BY-SA 3.0. https://commons.wikimedia.org/wiki/File:Lorenzini_pores_on_snout_of_tiger_shark.jpg.
Figure 3.11: An electric ray (Torpediniformes) showing locations of electric organs and electrocytes stacked within them. Kindred Grey. 2022. CC BY-SA 3.0. Includes Fish4345 – Flickr – NOAA Photo Library by NOAA, 2007 (public domain, https://commons.wikimedia.org/wiki/File:Fish4345_-_Flickr_-_NOAA_Photo_Library.jpg) and “Elektroplax Rochen,” by Alexander Graetz, 2006 (CC BY-SA 3.0, https://commons.wikimedia.org/wiki/File:Elektroplax_Rochen.png).
Figure 3.12: Andrij Z. Horodysky, PhD. Used with permission from Andrij Horodysky. Photo by Stjani Ben (May 2015; Thingvallavatn, Iceland). CC BY 4.0.
Text References
Bardach, J. E., and J. Atema. 1971. Handbook of sensory physiology, vol. 4: Chemical senses, part 2.: The sense of taste in fishes.
Bardach, J. E., J. H. Todd, and R. Crickmer. 1967. Orientation by taste in fish of the genus Ictalurus. Science 155:1276–1278.
Burton, D., and M. Burton. 2017. Perception and sensation: sensory cells, organs and systems. Pages 241–263 in D. Burton and M. Burton, Essential fish biology: diversity, structure, and function. Oxford University Press.
Collin, S. P., R. M. Kempster, and K. E. Yopak. 2016. How elasmobranchs sense their environment. Pages 19–99 in R. E. Shadwick, A. P. Farrell, and C. J. Brauner, editors. Fish Physiology 34(A: Physiology of elasmobranch fishes: structure and interaction with environment. Elsevier, Amsterdam.
Coombs, S., and J. Montgomery. 2014. The role of flow and the lateral line in the multisensory guidance of orienting behaviors. Pages 65–101 in H. Bleckmann et al., editors, Flow sensing in air and water, Springer-Verlag, Berlin and Heidelberg.
Cresci, A., C. M. Durif, C. B. Paris, S. D. Shema, A. B. Skiftesvik, and H. I. Browman. 2019. Glass eels (Anguilla anguilla) imprint the magnetic direction of tidal currents from their juvenile estuaries. Communications Biology 2:366. https://doi.org/10.1038/s42003-019-0619-8.
Dangles, O., D. Irschick, L. Chittka, and J. Casas. 2009. Variability in sensory ecology: expanding the bridge between physiology and evolutionary biology. Quarterly Review of Biology 84:51–74.
Dominoni, D. M., W. Halfwerk, E. Baird, R. T. Buxton, E. Fernández-Juricic, K. M. Fristrup, M. F. McKenna, D. J. Mennitt, E. K. Perkin, B. M. Seymoure, D. C. Stoner, J. B. Tennessen, C. A. Toth, L. P. Tyrrell, A. Wilson, C. D. Francis, N. H. Carter, and J. R. Barber. 2020. Why conservation biology can benefit from sensory ecology. Nature Ecology and Evolution 4:502–511. https://www.nature.com/articles/s41559-020-1135-4.
Douglas, R. H. and M. B. A. Djamgoz, editors. 1990. The visual system of fish. Chapman and Hall, London.
Draper, A. M., and M. J. Weissburg. 2019. Impacts of global warming and elevated CO2 on sensor behavior in predator-prey interactions: a review and synthesis. Frontiers in Ecology and Evolution 7:72. https://doi.org/10.3389/fevo.2019.00072.
Fisher, H. S., B. B. M. Wong, and G. G. Rosenthal. 2006. Alteration of the chemical environment disrupts communication in a freshwater fish. Proceedings of the Royal Society B 273:1187–1193.
Formicki, K., A. Korkzelecka-Orkisz, and A. Tanski. 2019. Magnetoreception in fish. Journal of Fish Biology 95:73–91.
Hamilton, S. L., N. S. Kasef, D. M. Stafford, E. G. Mattiasen, L. A. Kapphahn, C. A. Logan, E. P. Bjorkstedt, and S. M. Sogard. 2019. Ocean acidification and hypoxia can have opposite effects on rockfish otolith growth. Journal of Experimental Marine Biology and Ecology 521:151245. https://doi.org/10.1016/j.jembe.2019.151245.
Herrick, C. J. 1901. Nerves of siluroid fishes. Journal of Comparative Neurology 11:177–262.
Hoagland, H. 1933. Specific nerve impulses from gustatory and tactile receptors in catfish. Journal of General Physiology 16:685–693.
Kalmijn, A. J. 1971. The electric sense of sharks and rays. Journal of Experimental Biology 55:371–383.
Kamermans, M., and C. Hawryshyn. 2011. Teleost polarization vision: how it might work and what it might be good for. Philosophical Transactions of the Royal Society B 366:742–756.
Kasumyan, A. O. 2019. The taste system in fishes and the effects of environmental variables. Journal of Fish Biology 95:155–178.
King, B., and J. Long. 2020. How sharks and other animals evolved electroreception to find their prey. Phys Org website. Accesseded 18 May 2020. https://phys.org/news/2018-02-sharks-animals-evolved-electroreception-theirprey.html.
Konishi, J., and Y. Zotterman. 1961. Taste functions in the Carp: an electrophysiological study on gustatory fibres. Acta Physiological Scandinavica 52:150–161.
Kotrschal, K., and M. Palzenberger. 1992. Neuroecology of cyprinids: comparative, quantitative histology reveals diverse brain patterns. Environmental Biology of Fishes 33:135–152.
Kotrschal, K., M. J. van Staaden, and R. Huber. 1998. Fish brains: evolution and environmental relationships. Reviews in Fish Biology and Fisheries 8:373–408.
Kupprate, F., F. Hölker, and W. Kloas. 2020. Can skyglow reduce nocturnal melatonin concentrations in Eurasian Perch? Environmental Pollution 262:114324. https://doi.org/10.1016/j.envpol.2020.114324.
Li, L., and H. Maaswinkel. 2006. Visual sensitivity and signal processing in teleosts. Pages 179–241 in T. J. Hara and B. Zielinski, Sensory systems neuroscience, Elsevier Science & Technology, Amsterdam.
Liao, J. C. 2007. A review of fish swimming mechanics and behaviour in altered flows. Philosophical Transactions of the Royal Society of London B 362:1973–1993.
Mann, D. A., Z. Lu, and A. N. Popper.1997. A clupeid fish can detect ultrasound. Nature 389(6649):341–341. https://doi.org/10.1038/38636.
Marshall, N. J., F. Cortesi, F. de Busserolles, U. E. Siebeck, and K. L. Cheney. 2019. Colours and colour vision in reef fishes. Journal of Fish Biology 95:5–38.
Mayer, L. 2019. Sight fishing for trout. 2nd ed. Stackpole Books, Lanham, MD.
Merchant, N. D., M. J. Witt, P. Blondel, B. J. Godley, and G. H. Smith. 2012. Assessing sound exposure from shipping in coastal waters using a single hydrophone and automatic identification system (AIS) data. Marine Pollution Bulletin 64:1320–1329.
Mirjany M., T. Preuss, and D. S. Faber. 2011. Role of the lateral line mechanosensory system in directionality of Goldfish auditory evoked escape response. Journal of Experimental Biology 214:3358–3367.
Mogdans, J. 2019. Sensory ecology of the fish lateral-line system: morphological and physiological adaptations for the perception of hydrodynamic stimuli. Journal of Fish Biology 95:53–72.
Montgomery, J., H. Bleckmann, and S. Coombs. 2014. Sensory ecology and neuroethology of the lateral line. Pages 121–150 in S. Coombs et al., editors, The lateral line system, Springer, New York.
Montgomery, J. C., and A. J. Saunders. 1985. Functional morphology of the Piper Hyporhamphus ihi with reference to the role of the lateral line in feeding. Proceedings of the Royal Society of London B 224:197–208.
Neal, H. V., and H. W. Rand. 1939. Chordate anatomy. Blakiston’s Son, Philadelphia.
Newton, K. C., A. B. Gill, and S. M. Kajiura. 2019. Electroreception in marine fishes: chondrichthyans. Journal of Fish Biology 95:135–154.
Nicol, J. A. C. 1960. The biology of marine animals. Wiley Interscience, New York.
Nilsson, G. E. 1996. Brain and body oxygen requirements of Gnathonemus petersii, a fish with an exceptionally large brain. Journal of Experimental Biology 199:603–607.
Popper, A. N., A. D. Hawkins, O. Sand, and J. A. Sisneros. 2019. Examining the hearing abilities of fishes. Journal of the Acoustical Society of America 146:948–955.
Popper, A. N., A. D. Hawkins, and F. Thomsen. 2020. Taking the animal’s perspective regarding anthropogenic underwater sound. Trends in Ecology and Evolution 35(9):787–794. https://doi.org/10.1016/j.tree.2020.05.002.
Popper, A. N., and C. R. Schilt. 2008. Hearing and acoustic behavior: basic and applied considerations. Pages 17–48 in J. F. Webb, A. N. Popper, and R. R. Fay, editors, Fish bioacoustics, Springer handbook of auditory research 32, Springer, Berlin and Heidelberg.
Porteus, C. S., P. C. Hubbard, T. M. Uren Webster, R. van Aerle, A. V. M. Canário, E. M. Santos, and R. W. Wilson. 2018. Near-future CO2 levels impair the olfactory system of a marine fish. Nature Climate Change 8:737–743.
Preston, E. 2019. Lost at sea. Science 364(6446):1124–1127. DOI: 10.1126/science.364.6446.1124.
Putland, R. L., J. C. Montgomery, and C. A. Radford. 2019. Ecology of fish hearing. Journal of Fish Biology 95:39–52.
Richards, R. J., V. Raoult, D. M. Powter, and T. F. Gaston. 2018. Permanent magnets reduce bycatch of benthic sharks in an ocean trap fishery. Fisheries Research 208:16–21.
Siebek, U. E., A. N. Parker, D. Sprenger, L. M. Mäthger, and G. Wallis. 2010. A species of reef fish that uses ultraviolet patterns for covert face recognition. Current Biology 20:407–410.
Simpson, S. D., P. L. Munday, M. L. Wittenrich, R. Manassa, D. L. Dixson, M. Gagliano, and H. Y. Yan. 2011. Ocean acidification erodes a crucial auditory behavior in a marine fish. Global Change Biology 7:917–920. https://doi.org/10.1098/rsbl.2011.0293.
Sneddon, L. U. 2007. Sensory systems neuroscience. Vol. 25, pages 153–178 in T. J. Hara and B. Zielinski, editors, Fish physiology series, Elsevier, New York.
Stewart, T. A., and M. E. Hale. 2013. First description of a musculoskeletal linkage in an adipose fin: innovations for active control in a primitively passive appendage. Proceedings of the Royal Society B 280: 20122159. https://doi.org/10.1098/rspb.2012.2159.
Tavolga, W. N., A. N. Popper, and R. R. Fay. 1981. Hearing and sound communication in fishes. Springer-Verlag, New York.
Todd, J. H. 1971. The chemical languages of fishes. Scientific American 224(5):99–106.
Walls, G. L. 1942. The vertebrate eye and its adaptive radiation. Cranbrook Institute of Science Bulletin 19, Bloomfield Hills, MI (published as reprint in 1963 by Hafner, doi:10.5962/bhl.title.7369, see fig. 169, 577).
Zielinski, B. S. and T. J. Hara. 2006. Olfaction. Pages 1–43 in T. J. Hara and B. Zielinski, Sensory systems neuroscience, Elsevier Science & Technology, Amsterdam.
A sensory cell or sense organ, that responds to light falling on it
Tissue in the brain that secretes a hormone-like substance
A chemical substance produced and released into the environment by an animal, affecting the behavior or physiology of others of its species
Change direction when it enters at an angle
Regarding humankind as the central or most important element of existence, especially as opposed to God or animals
A countless or extremely great number
Short microscopic hairlike vibrating structures found in large numbers on the surface of certain cells causing currents in the surrounding fluid
The feeling or state of being full or satisfied