2 Basic Microbiology
2.1 Introduction to Prokaryotic Cells
All plant cells and animal cells are eukaryotic. Some microorganisms are composed of prokaryotic cells, whereas others are composed of eukaryotic cells. Prokaryotic microorganisms are classified within the domains Archaea and Bacteria, whereas eukaryotic organisms are classified within the domain Eukarya.
The structures inside a cell are analogous to the organs inside a human body, with unique structures suited to specific functions. Some of the structures found in prokaryotic cells are similar to those found in some eukaryotic cells; others are unique to prokaryotes. Although there are some exceptions, eukaryotic cells tend to be larger than prokaryotic cells. The comparatively larger size of eukaryotic cells dictates the need to compartmentalize various chemical processes within different areas of the cell, using complex membrane-bound organelles. In contrast, prokaryotic cells generally lack membrane-bound organelles; however, they often contain inclusions that compartmentalize their cytoplasm. Figure 2.1 illustrates structures typically associated with prokaryotic cells. These structures are described in more detail in the next section.
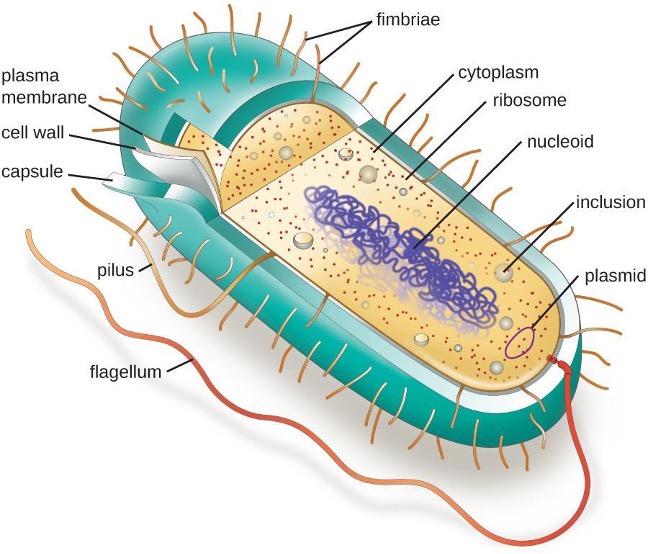
Common Cell Morphologies and Arrangements
Individual cells of a particular prokaryotic organism are typically similar in shape, or cell morphology. Although thousands of prokaryotic organisms have been identified, only a handful of cell morphologies are commonly seen microscopically. Table 2.1 names and illustrates cell morphologies commonly found in prokaryotic cells. In addition to cellular shape, prokaryotic cells of the same species may group together in certain distinctive arrangements depending on the plane of cell division. Some common arrangements are shown in table 2.2.
Name | Description | Illustration | Image |
---|---|---|---|
Coccus (pl. cocci) |
Round | ![]() |
![]() |
Bacillus (pl. bacilli) |
Rod | ![]() |
![]() |
Vibrio (pl. vibrios) |
Curved rod | ![]() |
![]() |
Coccobacillus (pl. coccobacilli) |
Short rod | ![]() |
![]() |
Spirillum (pl. spirilla) |
Spiral | ![]() |
![]() |
Spirochete (pl. spirochetes) |
Long, loose, helical spiral | ![]() |
![]() |
Table 2.1: Common prokaryotic cell shapes
Name | Description | Illustration |
---|---|---|
Coccus (pl. cocci) |
Single coccus | ![]() |
Diplococcus (pl. diplococci) |
Pair of two cocci | ![]() |
Tetrad (pl. tetrads) |
Grouping of four cells arranged in a square | ![]() |
Streptococcus (pl. streptococci) |
Chain of cocci | ![]() |
Staphylococcus (pl. staphylococci) |
Cluster of cocci | ![]() |
Bacillus (pl. bacilli) |
Single rod | ![]() |
Streptobacillus (pl. streptobacilli) |
Chain of rods | ![]() |
Table 2.2: Common prokaryotic cell arrangements
The Nucleoid
Prokaryotic DNA and DNA-associated proteins are concentrated within the nucleoid region of the cell (figure 2.2). In general, prokaryotic DNA interacts with nucleoid-associated proteins (NAPs) that assist in the organization and packaging of the chromosome. In bacteria, NAPs function similar to histones, which are the DNA-organizing proteins found in eukaryotic cells. In archaea, the nucleoid is organized by either NAPs or histone-like DNA organizing proteins.
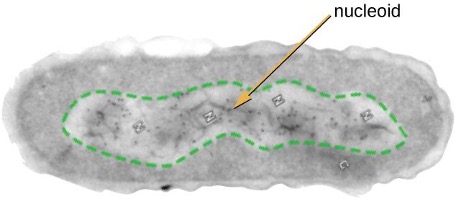
Plasmids
Prokaryotic cells may also contain extrachromosomal DNA, or DNA that is not part of the chromosome. This extrachromosomal DNA is found in plasmids, which are small, circular, double-stranded DNA molecules. Plasmids are more commonly found in bacteria; however, plasmids have been found in archaea and eukaryotic organisms. Plasmids often carry genes that confer advantageous traits such as antibiotic resistance. They are, therefore, important to the survival of the organism.
In prokaryotes, horizontal gene transfer (HGT), the introduction of genetic material from one organism to another organism within the same generation, is an important way to introduce genetic diversity. HGT allows even distantly related species to share genes, influencing their phenotypes. It is thought that HGT is more prevalent in prokaryotes but that only a small fraction of the prokaryotic genome may be transferred by this type of transfer at any one time. As the phenomenon is investigated more thoroughly, it may be revealed to be even more common. Many scientists believe that HGT and mutation are significant sources of genetic variation, the raw material for the process of natural selection, in prokaryotes.
HGT in prokaryotes is known to occur by the three primary mechanisms that are illustrated in figure 2.3 and summarized in table 2.3:
- Transformation: naked DNA is taken up from the environment
- Transduction: genes are transferred between cells in a virus (see section 2.11)
- Conjugation: use of a hollow tube called a conjugation pilus to transfer genes between cells
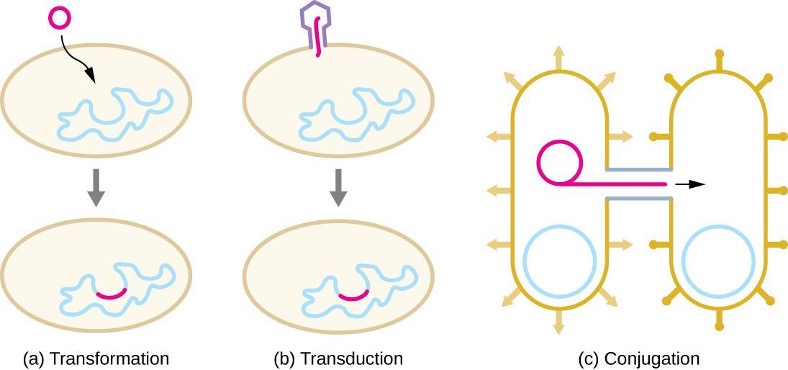
Term | Definition |
---|---|
Conjugation | Transfer of DNA through direct contact using a conjugation pilus |
Transduction | Mechanism of horizontal gene transfer in bacteria in which genes are transferred through viral infection |
Transformation | Mechanism of horizontal gene transfer in which naked environmental DNA is taken up by a bacterial cell |
Transposition | Process whereby DNA independently excises from one location in a DNA molecule and integrates elsewhere |
Table 2.3: Summary of mechanisms of genetic diversity in prokaryotes
Ribosomes
Ribosomes, themselves, are constructed from proteins, along with ribosomal RNA (rRNA). Prokaryotic ribosomes are found in the cytoplasm. They are called 70S ribosomes because they have a size of 70S (figure 2.4), whereas eukaryotic cytoplasmic ribosomes have a size of 80S. Although they are the same size, bacterial and archaeal ribosomes have different proteins and rRNA molecules, and the archaeal versions are more similar to their eukaryotic counterparts than to those found in bacteria.
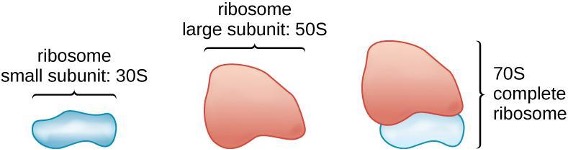
Inclusions
As single-celled organisms living in unstable environments, some prokaryotic cells have the ability to store excess nutrients within cytoplasmic structures called inclusions. Storing nutrients in a polymerized form is advantageous because it reduces the buildup of osmotic pressure that occurs as a cell accumulates solutes. Various types of inclusions store glycogen and starches, which contain carbon that cells can access for energy. Volutin granules, also called metachromatic granules because of their staining characteristics, are inclusions that store polymerized inorganic phosphate that can be used in metabolism and assist in the formation of biofilms. Microbes known to contain volutin granules include the archaea Methanosarcina, the bacterium Corynebacterium diphtheriae, and the unicellular eukaryotic alga Chlamydomonas. Sulfur granules, another type of inclusion, are found in sulfur bacteria of the genus Thiobacillus; these granules store elemental sulfur, which the bacteria use for metabolism.
Occasionally, certain types of inclusions are surrounded by a phospholipid monolayer embedded with protein. Polyhydroxybutyrate (PHB), which can be produced by species of Bacillus and Pseudomonas, is an example of an inclusion that displays this type of monolayer structure. Industrially, PHB has also been used as a source of biodegradable polymers for bioplastics. Several different types of inclusions are shown in figure 2.5.
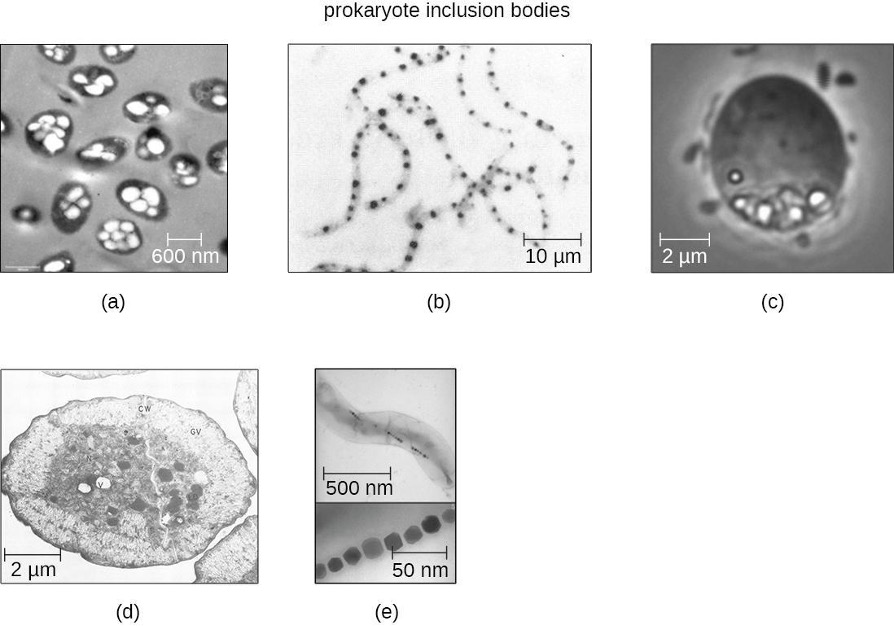
Endospores
Bacterial cells are generally observed as vegetative cells, but some genera of bacteria have the ability to form endospores, structures that essentially protect the bacterial genome in a dormant state when environmental conditions are unfavorable. Endospores (not to be confused with the reproductive spores formed by fungi) allow some bacterial cells to survive long periods without food or water, as well as exposure to chemicals, extreme temperatures, and even radiation. Table 2.4 compares the characteristics of vegetative cells and endospores.
Vegetative Cells | Endospores |
---|---|
Sensitive to extreme temperatures and radiation | Resistant to extreme temperatures and radiation |
Gram-positive | Do not absorb Gram stain, only special endospore stains (see section 2.2) |
Normal water content and enzymatic activity | Dehydrated; no metabolic activity |
Capable of active growth and metabolism | Dormant; no growth or metabolic activity |
Table 2.4: Characteristics of vegetative cells versus endospores
The process by which vegetative cells transform into endospores is called sporulation, and it generally begins when nutrients become depleted or environmental conditions become otherwise unfavorable (figure 2.6). The process begins with the formation of a septum in the vegetative bacterial cell. The septum divides the cell asymmetrically, separating a DNA forespore from the mother cell. The forespore, which will form the core of the endospore, is essentially a copy of the cell’s chromosomes and is separated from the mother cell by a second membrane. A cortex gradually forms around the forespore by laying down layers of calcium and dipicolinic acid between membranes. A protein spore coat then forms around the cortex while the DNA of the mother cell disintegrates. Further maturation of the endospore occurs with the formation of an outermost exosporium. The endospore is released upon disintegration of the mother cell, completing sporulation.
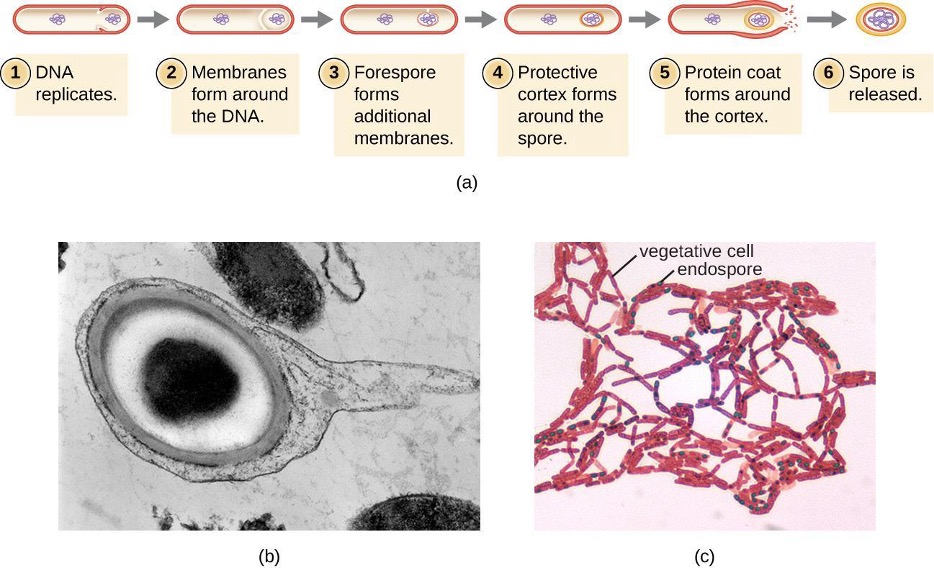
Not all bacteria have the ability to form endospores; however, there are a number of clinically significant endospore-forming, gram-positive bacteria of the genera Bacillus and Clostridium. These include B. anthracis, the causative agent of anthrax, which produces endospores capable of surviving for many decades1; C. tetani (causes tetanus); C. difficile (causes pseudomembranous colitis); C. perfringens (causes gas gangrene); and C. botulinum (causes botulism). Pathogens such as these are particularly difficult to combat because their endospores are so hard to kill.[1]
Cell Wall
The major component of bacterial cell walls is called peptidoglycan (or murein); it is only found in bacteria. Structurally, peptidoglycan resembles a layer of meshwork or fabric (figure 2.7). Each layer is composed of long chains of alternating molecules of N-acetylglucosamine (NAG) and N-acetylmuramic acid (NAM). The structure of the long chains has significant two-dimensional tensile strength due to the formation of peptide bridges that connect NAG and NAM within each peptidoglycan layer. In gram-negative bacteria, tetrapeptide chains extending from each NAM unit are directly cross-linked, whereas in gram-positive bacteria, these tetrapeptide chains are linked by pentaglycine cross-bridges. Peptidoglycan subunits are made inside of the bacterial cell and then exported and assembled in layers, giving the cell its shape.
Since peptidoglycan is unique to bacteria, many antibiotic drugs are designed to interfere with peptidoglycan synthesis, weakening the cell wall and making bacterial cells more susceptible to the effects of osmotic pressure (see section 9.2). In addition, certain cells of the human immune system are able to “recognize” bacterial pathogens by detecting peptidoglycan on the surface of a bacterial cell; these cells then engulf and destroy the bacterial cell, using enzymes such as lysozyme, which breaks down and digests the peptidoglycan in their cell walls (see section 1.6).
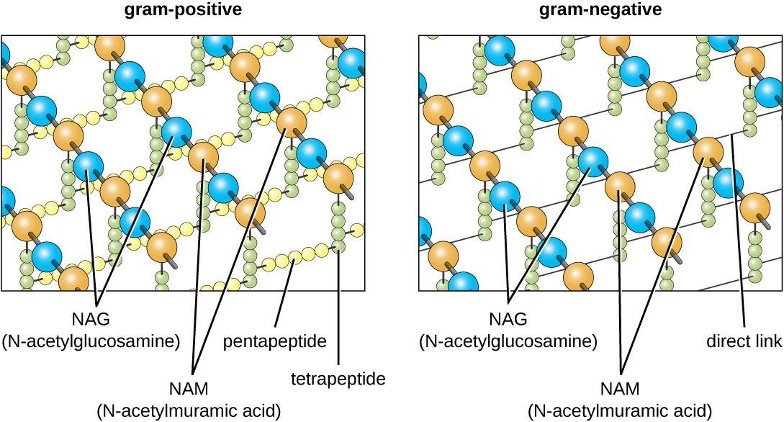
The Gram staining protocol (see section 2.2) is used to differentiate two common types of cell wall structures (figure 2.8). Gram-positive cells have a cell wall consisting of many layers of peptidoglycan totaling 30–100 nm in thickness. These peptidoglycan layers are commonly embedded with teichoic acids (TAs), carbohydrate chains that extend through and beyond the peptidoglycan layer.[2] TA is thought to stabilize peptidoglycan by increasing its rigidity. TA also plays a role in the ability of pathogenic gram-positive bacteria such as Streptococcus to bind to certain proteins on the surface of host cells, enhancing their ability to cause infection. In addition to peptidoglycan and TAs, bacteria of the family Mycobacteriaceae have an external layer of waxy mycolic acids in their cell wall; as described in section 2.2, these bacteria are referred to as acid-fast, since acid-fast stains must be used to penetrate the mycolic acid layer for purposes of microscopy (figure 2.9).
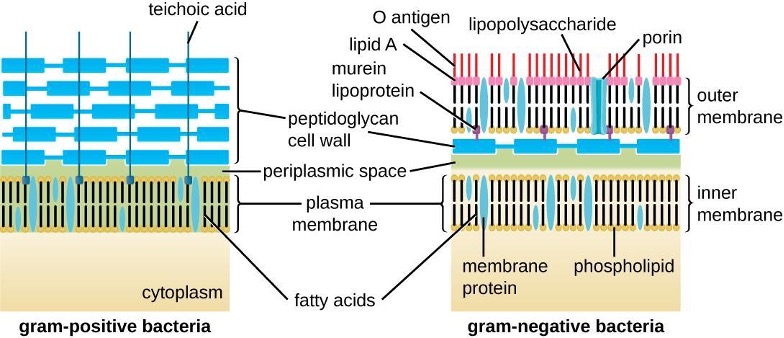
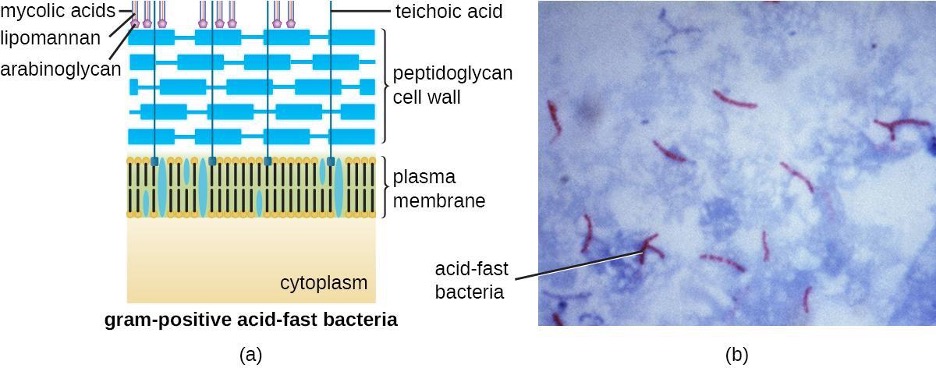
Gram-negative cells have a much thinner layer of peptidoglycan (no more than about 4 nm thick[3]) than gram-positive cells, and the overall structure of their cell envelope is more complex. In gram-negative cells, a gel-like matrix occupies the periplasmic space between the cell wall and the plasma membrane. Additionally, there is a second lipid bilayer called the outer membrane, which is external to the peptidoglycan layer (figure 2.8). This outer membrane is attached to the peptidoglycan by murein lipoprotein. The outer leaflet of the outer membrane contains the molecule lipopolysaccharide (LPS), which functions as an endotoxin in infections involving gram-negative bacteria, contributing to symptoms such as fever, hemorrhaging, and septic shock. Each LPS molecule is composed of Lipid A, a core polysaccharide, and an O-side chain that is composed of sugar-like molecules that comprise the external face of the LPS (figure 2.10). The composition of the O-side chain varies between different species and strains of bacteria. Parts of the O-side chain called antigens can be detected using serological or immunological tests to identify specific pathogenic strains like Escherichia coli O157:H7, a deadly strain of bacteria that causes bloody diarrhea and kidney failure.
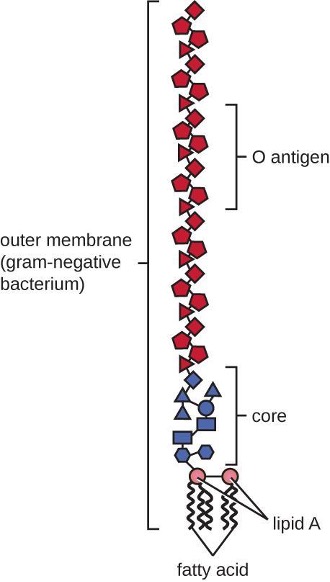
Archaeal cell wall structure differs from that of bacteria in several significant ways. First, archaeal cell walls do not contain peptidoglycan; instead, they contain a similar polymer called pseudopeptidoglycan (pseudomurein) in which NAM is replaced with a different subunit. Other archaea may have a layer of glycoproteins or polysaccharides that serves as the cell wall instead of pseudopeptidoglycan. Last, as is the case with some bacterial species, there are a few archaea that appear to lack cell walls entirely.
Glycocalyces and S-Layers
Although most prokaryotic cells have cell walls, some may have additional cell envelope structures exterior to the cell wall, such as glycocalyces and S-layers. A glycocalyx is a sugar coat, of which there are two important types: capsules and slime layers. A capsule is an organized layer located outside of the cell wall and usually composed of polysaccharides or proteins (figure 2.11). A slime layer is a less tightly organized layer that is only loosely attached to the cell wall and can be more easily washed off. Slime layers may be composed of polysaccharides, glycoproteins, or glycolipids.
Glycocalyces allow cells to adhere to surfaces, aiding in the formation of biofilms (colonies of microbes that form in layers on surfaces). In nature, most microbes live in mixed communities within biofilms, partly because the biofilm affords them some level of protection. Biofilms generally hold water like a sponge, preventing desiccation. They also protect cells from predation and hinder the action of antibiotics and disinfectants. All of these properties are advantageous to the microbes living in a biofilm, but they present challenges in a clinical setting, where the goal is often to eliminate microbes.
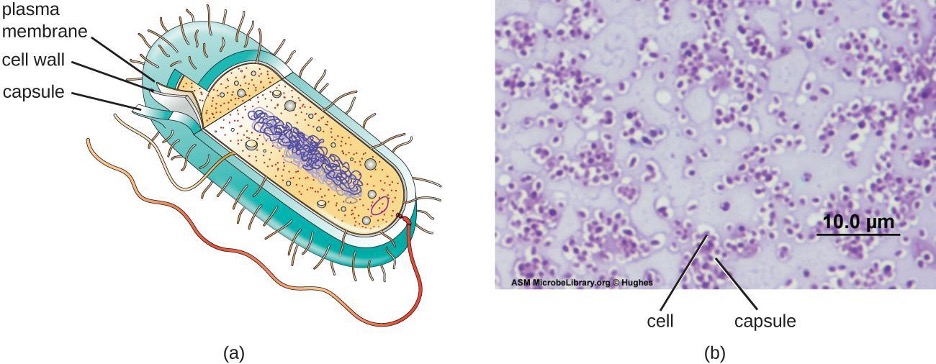
The ability to produce a capsule can contribute to a microbe’s pathogenicity (ability to cause disease) because the capsule can make it more difficult for phagocytic cells (such as white blood cells) to engulf and kill the microorganism. Streptococcus pneumoniae, for example, produces a capsule that is well known to aid in this bacterium’s pathogenicity. As explained in section 2.2, capsules are difficult to stain for microscopy; negative staining techniques are typically used.
An S-layer is another type of cell envelope structure; it is composed of a mixture of structural proteins and glycoproteins. In bacteria, S-layers are found outside the cell wall, but in some archaea, the S-layer serves as the cell wall. The exact function of S-layers is not entirely understood, and they are difficult to study. Available evidence suggests that they may play a variety of functions in different prokaryotic cells, such as helping the cell withstand osmotic pressure and, for certain pathogens, interacting with the host immune system.
Filamentous Appendages
Many bacterial cells have protein appendages embedded within their cell envelopes that extend outward, allowing interaction with the environment. These appendages can attach to other surfaces, transfer DNA, or provide movement. Filamentous appendages include fimbriae, pili, and flagella.
Fimbriae and Pili
Fimbriae and pili are structurally similar and, because differentiation between the two is problematic, these terms are often used interchangeably.[4][5] The term fimbriae commonly refers to short bristle-like proteins projecting from the cell surface by the hundreds. Fimbriae enable a cell to attach to surfaces and to other cells. For pathogenic bacteria, adherence to host cells is important for colonization, infectivity, and virulence. Adherence to surfaces is also important in biofilm formation.
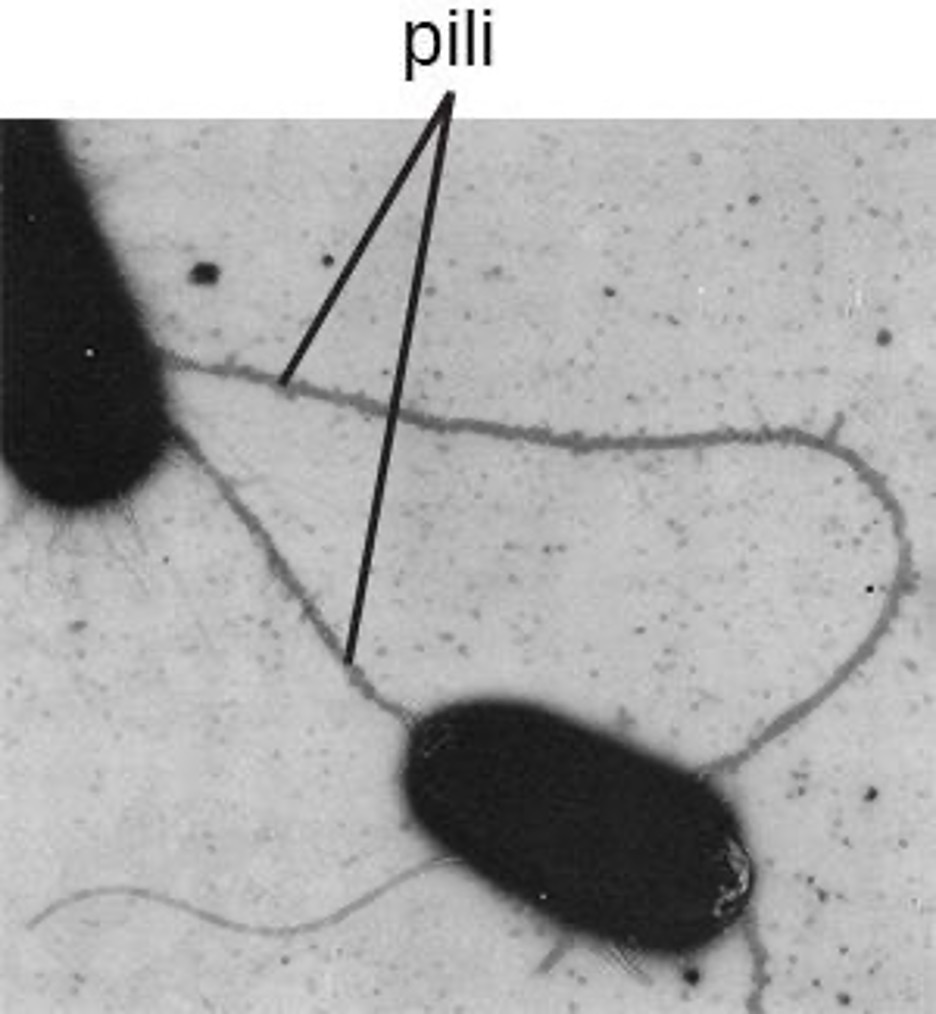
The term pili (singular: pilus) commonly refers to longer, less numerous protein appendages that aid in attachment to surfaces (figure 2.12). A specific type of pilus, called the F pilus or sex pilus, is important in the transfer of DNA between bacterial cells, which occurs between members of the same generation when two cells physically transfer or exchange parts of their respective genomes.
Flagella
Bacterial flagella act like propellers. They are stiff spiral filaments composed of flagellin protein subunits that extend outward from the cell and spin in solution. The basal body is the motor for the flagellum and is embedded in the plasma membrane (figure 2.13). A hook region connects the basal body to the filament. Gram-positive and gram-negative bacteria have different basal body configurations due to differences in cell wall structure.
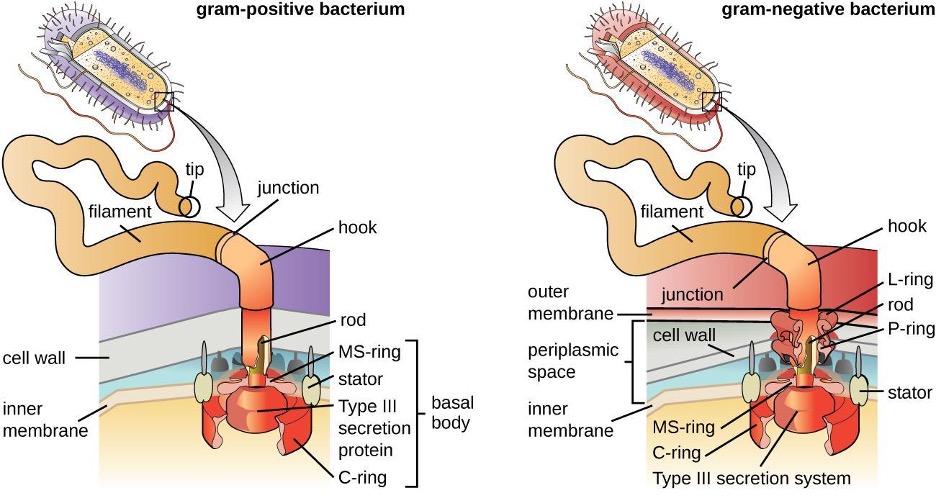
Different types of motile bacteria exhibit different arrangements of flagella (figure 2.14). A bacterium with a singular flagellum, typically located at one end of the cell (polar), is said to have a monotrichous flagellum. An example of a monotrichously flagellated bacterial pathogen is Vibrio cholerae, the gram-negative bacterium that causes cholera. Cells with amphitrichous flagella have a flagellum or tufts of flagella at each end. An example is Spirillum minor, the cause of spirillary (Asian) rat-bite fever or sodoku. Cells with lophotrichous flagella have a tuft at one end of the cell. The gram-negative bacillus Pseudomonas aeruginosa, an opportunistic pathogen known for causing many infections including swimmer’s ear and burn wound infections, has lophotrichous flagella. Flagella that cover the entire surface of a bacterial cell are called peritrichous flagella. The gram-negative bacterium E. coli shows a peritrichous arrangement of flagella.

Directional movement depends on the configuration of the flagella. Bacteria can move in response to a variety of environmental signals, including light (phototaxis), magnetic fields (magnetotaxis) using magnetosomes, and, most commonly, chemical gradients (chemotaxis). In a peritrichous bacterium, the flagella are all bundled together in a notably streamlined way (figure 2.15), allowing for efficient movement.
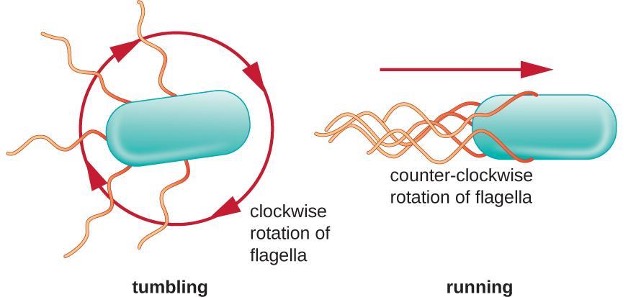
2.2 Staining Microscopic Specimens
Gram Staining
The Gram stain procedure is a differential staining procedure that involves multiple steps. It was developed by Danish microbiologist Hans Christian Gram in 1884 as an effective method to distinguish between bacteria with different types of cell walls. Even today it remains one of the most frequently used staining techniques. The steps of the Gram stain procedure are listed below and illustrated in table 2.5 and 2.6.
- First, crystal violet, a primary stain, is applied to a heat-fixed smear, giving all of the cells a purple color.
- Next, Gram’s iodine, a mordant, is added. A mordant is a substance used to set or stabilize stains or dyes; in this case, Gram’s iodine acts like a trapping agent that complexes with the crystal violet, making the crystal violet–iodine complex clump and stay contained in thick layers of peptidoglycan in the cell walls.
- Next, a decolorizing agent is added, usually ethanol or an acetone/ethanol solution. Cells that have thick peptidoglycan layers in their cell walls are much less affected by the decolorizing agent; they generally retain the crystal violet dye and remain purple. However, the decolorizing agent more easily washes the dye out of cells with thinner peptidoglycan layers, making them again colorless.
- Finally, a secondary counterstain, usually safranin, is added. This stains the decolorized cells pink and is less noticeable in the cells that still contain the crystal violet dye.
The purple, crystal-violet stained cells are referred to as gram-positive cells, while the red, safranin-dyed cells are gram-negative (figure 2.16). Besides their differing interactions with dyes and decolorizing agents, the chemical differences between gram-positive and gram-negative cells have other implications with clinical relevance. For example, Gram staining can help clinicians classify bacterial pathogens in a sample into categories associated with specific properties. Gram-negative bacteria tend to be more resistant to certain antibiotics than gram-positive bacteria. We will discuss this and other applications of Gram staining in more detail later.
Gram staining steps | Cells effects | Gram-positive | Gram-negative |
---|---|---|---|
Step 1 Crystal violet primary stain added to specimen smear |
Stains cells purple or blue. | ![]() |
![]() |
Step 2 Iodine mordant makes dye less soluble so it adheres to cell walls. |
Stains cells purple or blue. | ![]() |
![]() |
Step 3 Alcohol decolorizer washes away stain from gram-negative cell walls. |
Gram-positive cells remain purple or blue. Gram-negative cells are colorless. | ![]() |
![]() |
Step 4 Safranin counterstain allows dye adherence to gram-negative cells. |
Gram-positive cells remain purple or blue. Gram-negative cells appear pink or red. | ![]() |
![]() |
Table 2.5: Gram staining process. Gram-staining is a differential staining technique that uses a primary stain and a secondary counterstain to distinguish between gram-positive and gram-negative bacteria.
Acid-Fast Stains
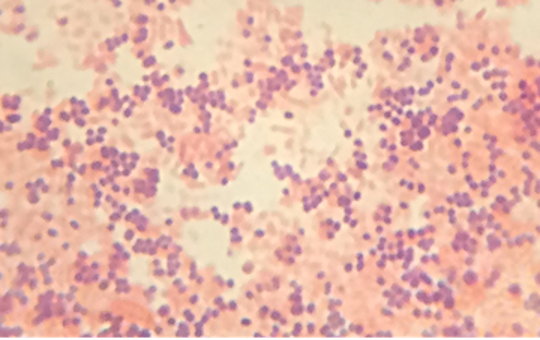
Acid-fast staining (tables 2.5 and 2.6) is another commonly used, differential staining technique that can be an important diagnostic tool. An acid-fast stain is able to differentiate two types of gram-positive cells: those that have waxy mycolic acids in their cell walls and those that do not. Two different methods for acid-fast staining are the Ziehl-Neelsen technique and the Kinyoun technique. Both use carbolfuchsin as the primary stain. The waxy, acid-fast cells retain the carbolfuchsin even after a decolorizing agent (an acid-alcohol solution) is applied. A secondary counterstain, methylene blue, is then applied, which renders non–acid-fast cells blue.
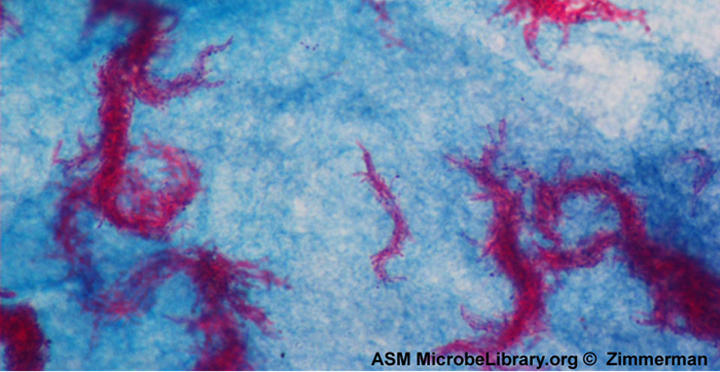
Mycobacterium tuberculosis, the bacterium that causes tuberculosis, can be detected in specimens based on the presence of acid-fast bacilli. Often, a smear is prepared from a sample of the patient’s sputum and then stained using the Ziehl-Neelsen technique (figure 2.17). If acid-fast bacteria are confirmed, they are generally cultured to make a positive identification. Variations of this approach can be used as a first step in determining whether M. tuberculosis or other acid-fast bacteria are present, though samples from elsewhere in the body (such as urine) may contain other Mycobacterium species (figure 2.42).
An alternative approach for determining the presence of M. tuberculosis is immunofluorescence. In this technique, fluorochrome-labeled antibodies bind to M. tuberculosis, if present. Antibody-specific fluorescent dyes can be used to view the mycobacteria with a fluorescence microscope.
Capsule Staining
Certain bacteria and yeasts have a protective outer structure called a capsule. Since the presence of a capsule is directly related to a microbe’s virulence (its ability to cause disease), the ability to determine whether cells in a sample have capsules is an important diagnostic tool. Capsules do not absorb most basic dyes; therefore, a negative staining technique (staining around the cells) is typically used for capsule staining. The dye stains the background but does not penetrate the capsules, which appear like halos around the borders of the cell. The specimen does not need to be heat-fixed prior to negative staining.
One common negative staining technique for identifying encapsulated yeast and bacteria is to add a few drops of India ink or nigrosin to a specimen. Other capsular stains can also be used to negatively stain encapsulated cells (figure 2.18). Alternatively, positive and negative staining techniques can be combined to visualize capsules: The positive stain colors the body of the cell, and the negative stain colors the background but not the capsule, leaving a halo around each cell.
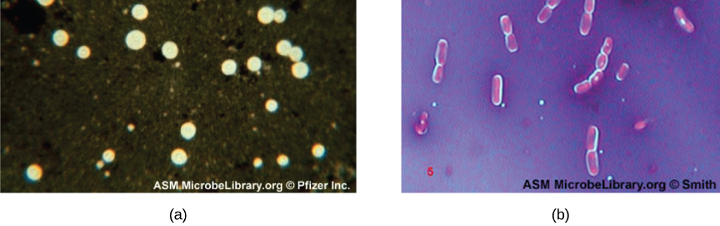
Endospore Staining
Endospores are structures produced within certain bacterial cells that allow them to survive harsh conditions. Gram staining alone cannot be used to visualize endospores, which appear clear when Gram-stained cells are viewed. Endospore staining uses two stains to differentiate endospores from the rest of the cell. The Schaeffer-Fulton method (the most commonly used endospore-staining technique) uses heat to permeabilize the cortex and protein spore coat, allowing the primary stain to penetrate into the endospore. Washing with water decolorizes the cell, but the endospore retains the green stain. The cell is then counterstained pink with safranin. The resulting image reveals the shape and location of endospores, if they are present. The green endospores will appear either within the pink vegetative cells or as separate from the pink cells altogether. If no endospores are present, then only the pink vegetative cells will be visible (figure 2.19).
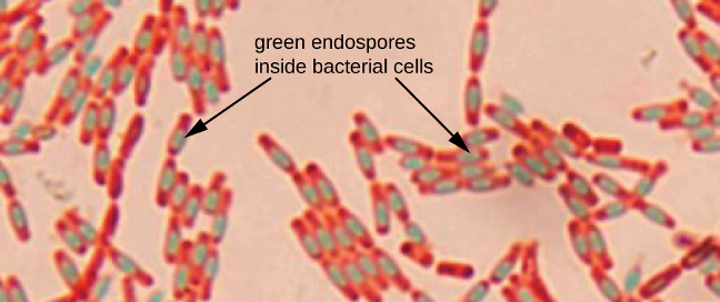
Endospore-staining techniques are important for identifying Bacillus and Clostridium, two genera of endospore-producing bacteria that contain clinically significant species. Among others, B. anthracis (which causes anthrax) has been of particular interest because of concern that its spores could be used as a bioterrorism agent. C. difficile is a particularly important species responsible for the typically hospital-acquired infection known as “C. diff.”
Flagella Staining
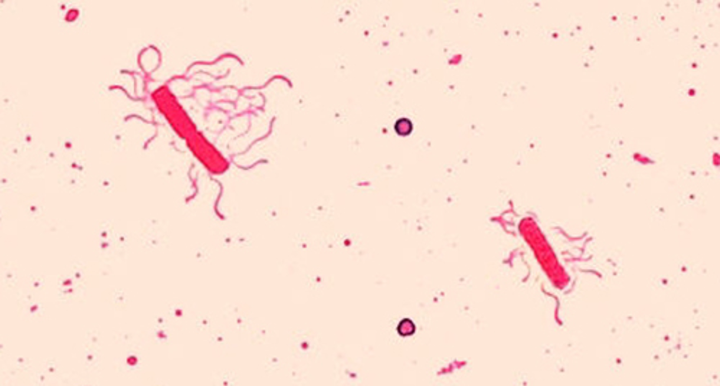
Flagella (singular: flagellum) are tail-like cellular structures used for locomotion by some bacteria, archaea, and eukaryotes. Because they are so thin, flagella typically cannot be seen under a light microscope without a specialized flagella staining technique. Flagella staining thickens the flagella by first applying mordant (generally tannic acid, but sometimes potassium alum), which coats the flagella; then the specimen is stained with pararosaniline (most commonly) or basic fuchsin (figure 2.20).
Though flagella staining is uncommon in clinical settings, the technique is commonly used by microbiologists, since the location and number of flagella can be useful in classifying and identifying bacteria in a sample.
Stain Type | Specific Dyes | Purpose | Outcome | Sample Images |
---|---|---|---|---|
Basic stains | Methylene blue, crystal violet, malachite green, basic fuchsin, carbolfuchsin, safranin | Stain negatively charged molecules and structures such as nucleic acids and proteins | Positive stain | ![]() |
Acidic stains | Eosin, acid fuchsin, rose bengal, Congo red | Stain positively charged molecules and structures such as proteins | Can be either a positive or negative stain, depending on the cells chemistry | ![]() |
Negative stains | India ink, nigrosin | Stains background, not specimen | Dark background with light specimen | ![]() |
Table 2.6: Simple stains.
2.3 How Microbes Grow
The bacterial cell cycle involves the formation of new cells through the replication of DNA and partitioning of cellular components into two daughter cells. In prokaryotes, reproduction is always asexual, although extensive genetic recombination in the form of horizontal gene transfer takes place. Most bacteria have a single circular chromosome; however, some exceptions exist. For example, Borrelia burgdorferi, the causative agent of Lyme disease, has a linear chromosome.
Binary Fission
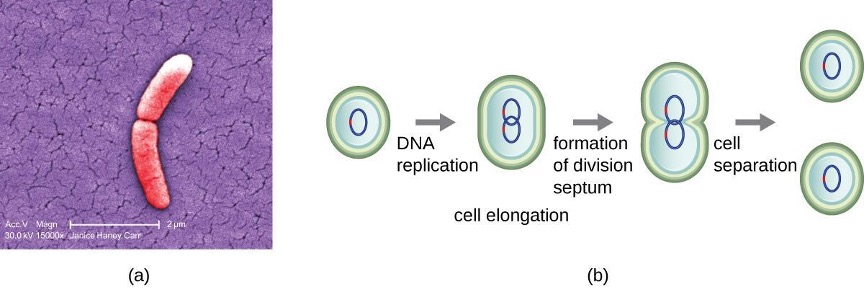
The most common mechanism of cell replication in bacteria is a process called binary fission, which is depicted in figure 2.21. Before dividing, the cell grows and increases its number of cellular components. Next, the replication of DNA starts at a location on the circular chromosome called the origin of replication, where the chromosome is attached to the inner cell membrane. Replication continues in opposite directions along the chromosome until the terminus is reached.
The Growth Curve
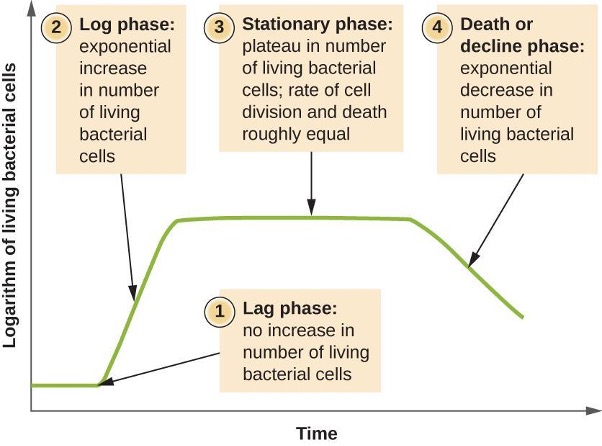
Microorganisms grown in a closed culture (also known as a batch culture), in which no nutrients are added and most waste is not removed, follow a reproducible growth pattern referred to as the growth curve. The culture density is defined as the number of cells per unit volume. In a closed environment, the culture density is also a measure of the number of cells in the population. Infections of the body do not always follow the growth curve, but correlations can exist depending upon the site and type of infection. When the number of live cells is plotted against time, distinct phases can be observed in the curve (figure 2.22).
The Lag Phase
The beginning of the growth curve represents a small number of cells, referred to as an inoculum, that are added to a fresh culture medium, a nutritional broth that supports growth. The initial phase of the growth curve is called the lag phase, during which cells are gearing up for the next phase of growth. The number of cells does not change during the lag phase; however, cells grow larger and are metabolically active, synthesizing proteins needed to grow within the medium. If any cells were damaged or shocked during the transfer to the new medium, repair takes place during the lag phase. The duration of the lag phase is determined by many factors, including the species and genetic make-up of the cells, the composition of the medium, the temperature of the medium, and the size of the original inoculum.
The Log Phase
In the logarithmic (log) growth phase, sometimes called the exponential growth phase, the cells are actively dividing by binary fission and their number increases exponentially. For any given bacterial species, the generation time under specific growth conditions (nutrients, temperature, pH, and so forth) is genetically determined, and this generation time is called the intrinsic growth rate. During the log phase, the relationship between time and number of cells is not linear but exponential; however, the growth curve is often plotted on a semilogarithmic graph, as shown in figure 2.23, which gives the appearance of a linear relationship.
Cells in the log phase show constant growth rate and uniform metabolic activity. For this reason, cells in the log phase are preferentially used for industrial applications and research work. The log phase is also the stage where bacteria are the most susceptible to the action of disinfectants and common antibiotics that affect protein, DNA, and cell-wall synthesis.
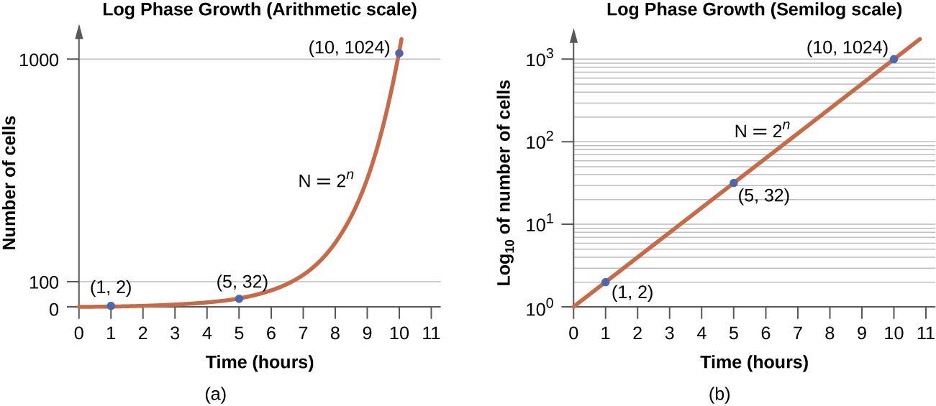
Stationary Phase
As the number of cells increases through the log phase, several factors contribute to a slowing of the growth rate. Waste products accumulate and nutrients are gradually used up. In addition, gradual depletion of oxygen begins to limit aerobic cell growth. This combination of unfavorable conditions slows and finally stalls population growth. The total number of live cells reaches a plateau referred to as the stationary phase (figure 2.22). In this phase, the number of new cells created by cell division is now equivalent to the number of cells dying; thus, the total population of living cells is relatively stagnant. The culture density in a stationary culture is constant. The culture’s carrying capacity, or maximum culture density, depends on the types of microorganisms in the culture and the specific conditions of the culture; however, carrying capacity is constant for a given organism grown under the same conditions.
During the stationary phase, cells switch to a survival mode of metabolism. As growth slows, so too does the synthesis of peptidoglycans, proteins, and nucleic-acids; thus, stationary cultures are less susceptible to antibiotics that disrupt these processes. In bacteria capable of producing endospores, many cells undergo sporulation during the stationary phase. Secondary metabolites, including antibiotics, are synthesized in the stationary phase. In certain pathogenic bacteria, the stationary phase is also associated with the expression of virulence factors, products that contribute to a microbe’s ability to survive, reproduce, and cause disease in a host organism. For example, quorum sensing in Staphylococcus aureus initiates the production of enzymes that can break down human tissue and cellular debris, clearing the way for bacteria to spread to new tissue where nutrients are more plentiful.
The Death Phase
As a culture medium accumulates toxic by-products and nutrients are exhausted, cells die in greater and greater numbers. Soon, the number of dying cells exceeds the number of dividing cells, leading to an exponential decrease in the number of cells (figure 2.22). This is the aptly named death phase, sometimes called the decline phase. Many cells lyse and release nutrients into the medium, allowing surviving cells to maintain viability and form endospores. A few cells, the so-called persisters, are characterized by a slow metabolic rate. Persister cells are medically important because they are associated with certain chronic infections, such as tuberculosis, that do not respond to antibiotic treatment.
2.4 Proteobacteria
In 1987, the American microbiologist Carl Woese (1928–2012) suggested that a large and diverse group of bacteria that he called “purple bacteria and their relatives” should be defined as a separate phylum within the domain Bacteria based on the similarity of the nucleotide sequences in their genome.[6] This phylum of gram-negative bacteria subsequently received the name Proteobacteria. It includes many bacteria that are part of the normal human microbiota as well as many pathogens. The Proteobacteria are further divided into five classes: Alphaproteobacteria, Betaproteobacteria, Gammaproteobacteria, Deltaproteobacteria, and Epsilonproteobacteria.
Alphaproteobacteria
The first class of Proteobacteria is the Alphaproteobacteria, many of which are obligate or facultative intracellular bacteria. Some species are characterized as oligotrophs, organisms capable of living in low-nutrient environments such as deep oceanic sediments, glacial ice, or deep undersurface soil.
Among the Alphaproteobacteria are rickettsias, obligate intracellular pathogens, that require part of their life cycle to occur inside other cells called host cells. When not growing inside a host cell, Rickettsia are metabolically inactive outside the host cell. They cannot synthesize their own adenosine triphosphate (ATP), and, therefore, rely on cells for their energy needs.
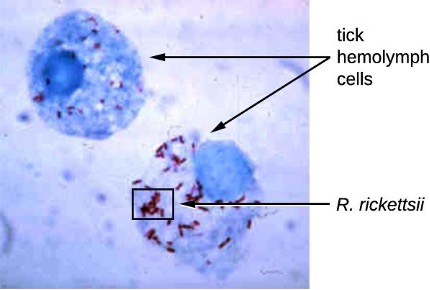
Rickettsia spp. include a number of serious human pathogens. For example, R. rickettsii causes Rocky Mountain spotted fever, a life-threatening form of meningoencephalitis (inflammation of the membranes that wrap the brain). R. rickettsii infects ticks and can be transmitted to humans via a bite from an infected tick (figure 2.24).
Another species of Rickettsia, R. prowazekii, is spread by lice. It causes epidemic typhus, a severe infectious disease common during warfare and mass migrations of people. R. prowazekii infects human endothelial cells, causing inflammation of the inner lining of blood vessels, high fever, abdominal pain, and sometimes delirium. A relative, R. typhi, causes a less severe disease known as murine or endemic typhus, which is still observed in the southwestern United States during warm seasons.
Table 2.7 summarizes the characteristics of important genera of Alphaproteobacteria.
Genus | Microscopic Morphology | Unique Characteristics |
---|---|---|
Agrobacterium | Gram-negative bacillus | Plant pathogen; one species, A. tumefaciens, causes tumors in plants |
Bartonella | Gram-negative, pleomorphic, flagellated coccobacillus | Facultative intracellular bacteria, transmitted by lice and fleas, cause trench fever and cat scratch disease in humans |
Brucella | Gram-negative, small, flagellated coccobacillus | Facultative intracellular bacteria, transmitted by contaminated milk from infected cows, cause brucellosis in cattle and humans |
Caulobacter | Gram-negative bacillus | Used in studies on cellular adaptation and differentiation because of its peculiar life cycle (during cell division, forms swarm cells and stalked cells) |
Coxiella | Small, gram-negative bacillus | Obligatory intracellular bacteria; cause Q fever; potential for use as biological weapon |
Ehrlichia | Very small, gram-negative, coccoid or ovoid bacteria | Obligatory intracellular bacteria; can be transported from cell to cell; transmitted by ticks; cause ehrlichiosis (destruction of white blood cells and inflammation) in humans and dogs |
Hyphomicrobium | Gram-negative bacilli; grows from a stalk | Similar to Caulobacter (above) |
Methylocystis | Gram-negative, coccoid or short bacilli | Nitrogen-fixing aerobic bacteria |
Rhizobium | Gram-negative, rectangular bacilli with rounded ends forming clusters | Nitrogen-fixing bacteria that live in soil and form symbiotic relationship with roots of legumes (e.g., clover, alfalfa, and beans) |
Rickettsia | Gram-negative, highly pleomorphic bacteria (may be cocci, rods, or threads) | Obligate intracellular bacteria; transmitted by ticks; may cause Rocky Mountain spotted fever and typhus |
Table 2.7: Class Alphaproteobacteria
Betaproteobacteria
Betaproteobacteria are a diverse group of bacteria. The different bacterial species within this group utilize a wide range of metabolic strategies and can survive in a range of environments. Some genera include species that are human pathogens, able to cause severe, sometimes life-threatening disease. The genus Neisseria, for example, includes the bacteria N. gonorrhoeae, the causative agent of the STI gonorrhea, and N. meningitides, the causative agent of bacterial meningitis.
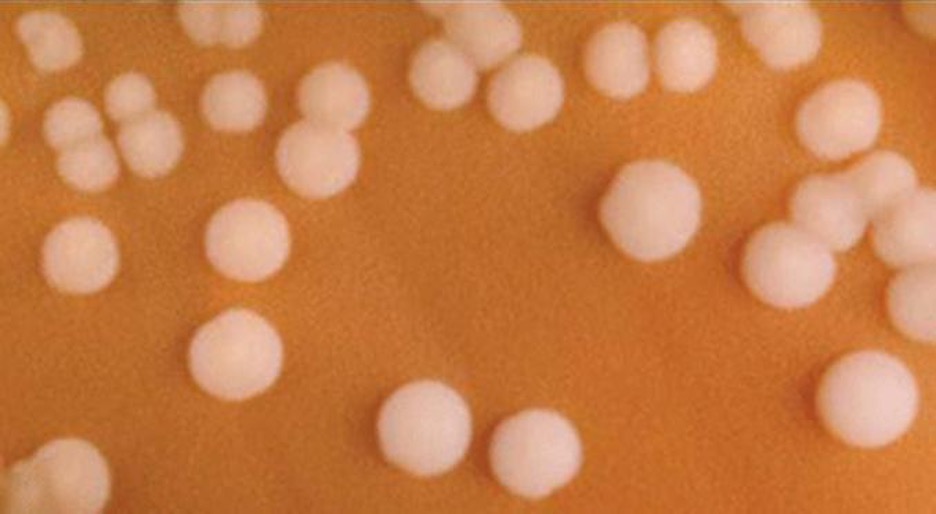
Neisseria are cocci that live on mucosal surfaces of the human body. They are fastidious, being difficult to culture as well as requiring high levels of moisture, nutrient supplements, and carbon dioxide. Also, Neisseria are microaerophilic, meaning that they require low levels of oxygen. For optimal growth and for the purposes of identification, Neisseria spp. are grown on chocolate agar (i.e., agar supplemented by partially hemolyzed red blood cells). Their characteristic pattern of growth in culture is diplococcal: pairs of cells resembling coffee beans (figure 2.25).
The pathogen responsible for pertussis (whooping cough) is also a member of Betaproteobacteria. The bacterium Bordetella pertussis, from the order Burkholderiales, produces several toxins that paralyze the movement of cilia in the human respiratory tract and directly damage cells of the respiratory tract, causing a severe cough.
Table 2.8 summarizes the characteristics of important genera of Betaproteobacteria.
Example Genus | Microscopic Morphology | Unique Characteristics |
---|---|---|
Bordetella | A small, gram-negative coccobacillus | Aerobic, very fastidious; B. pertussis causes pertussis (whooping cough) |
Burkholderia | Gram-negative bacillus | Aerobic, aquatic, cause diseases in horses and humans (especially patients with cystic fibrosis); agents of nosocomial infections |
Leptothrix | Gram-negative, sheathed, filamentous bacillus | Aquatic; oxidize iron and manganese; can live in wastewater treatment plants and clog pipes |
Neisseria | Gram-negative, coffee bean-shaped coccus forming pairs | Require moisture and high concentration of carbon dioxide; oxidase positive, grow on chocolate agar; pathogenic species cause gonorrhea and meningitis |
Thiobacillus | Gram-negative bacillus | Thermophilic, acidophilic, strictly aerobic bacteria; oxidize iron and sulfur |
Table 2.8: Class Betaproteobacteria
Gammaproteobacteria
The most diverse class of gram-negative bacteria is Gammaproteobacteria, and it includes a number of human pathogens. For example, a large and diverse family, Pseudomonaceae, includes the genus Pseudomonas. Within this genus is the species P. aeruginosa, a pathogen responsible for diverse infections in various regions of the body. P. aeruginosa is a strictly aerobic, nonfermenting, highly motile bacterium. It often infects wounds and burns, can be the cause of chronic urinary tract infections, and can be an important cause of respiratory infections in patients with cystic fibrosis or patients on mechanical ventilators. Infections by P. aeruginosa are often difficult to treat because the bacterium is resistant to many antibiotics and has a remarkable ability to form biofilms. Other representatives of Pseudomonas include the fluorescent (glowing) bacterium P. fluorescens and the soil bacteria P. putida, which is known for its ability to degrade xenobiotics (substances not naturally produced or found in living organisms).
The Pasteurellaceae also includes several clinically relevant genera and species. This family includes several bacteria that are human and/or animal pathogens. For example, Pasteurella haemolytica causes severe pneumonia in sheep and goats. P. multocida is a species that can be transmitted from animals to humans through bites, causing infections of the skin and deeper tissues. The genus Haemophilus contains two human pathogens, H. influenzae and H. ducreyi. Despite its name, H. influenzae does not cause influenza (which is a viral disease). H. influenzae can cause both upper and lower respiratory tract infections, including sinusitis, bronchitis, ear infections, and pneumonia. Before the development of effective vaccination, strains of H. influenzae were a leading cause of more invasive diseases, like meningitis in children. H. ducreyi causes the STI known as chancroid.
The order Vibrionales includes the human pathogen Vibrio cholerae. This comma-shaped aquatic bacterium thrives in highly alkaline environments like shallow lagoons and sea ports. A toxin produced by V. cholerae causes hypersecretion of electrolytes and water in the large intestine, leading to profuse watery diarrhea and dehydration. V. parahaemolyticus is also a cause of gastrointestinal disease in humans, whereas V. vulnificus causes serious and potentially life-threatening cellulitis (infection of the skin and deeper tissues) and blood-borne infections. Another representative of Vibrionales, Aliivibrio fischeri, engages in a symbiotic relationship with squid. The squid provides nutrients for the bacteria to grow and the bacteria produce bioluminescence that protects the squid from predators (figure 2.26).
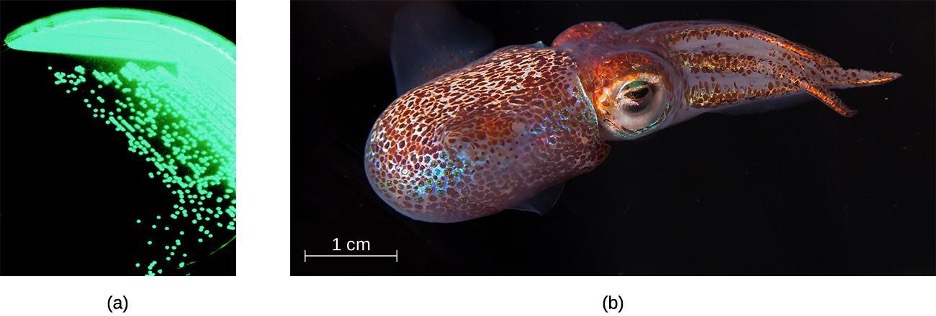
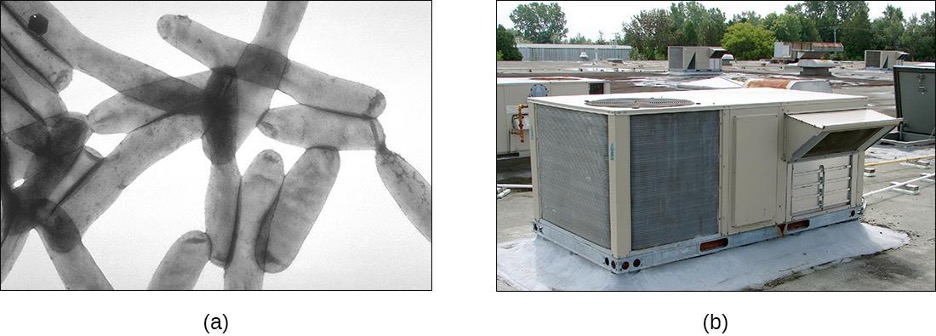
The genus Legionella also belongs to the Gammaproteobacteria. L. pneumophila, the pathogen responsible for Legionnaires disease, is an aquatic bacterium that tends to inhabit pools of warm water, such as those found in the tanks of air conditioning units in large buildings (figure 2.27). Because the bacteria can spread in aerosols, outbreaks of Legionnaires disease often affect residents of a building in which the water has become contaminated with Legionella. In fact, these bacteria derive their name from the first known outbreak of Legionnaires disease, which occurred in a hotel hosting an American Legion veterans’ association convention in Philadelphia in 1976.
Enterobacteriaceae is a large family of enteric (intestinal) bacteria belonging to the Gammaproteobacteria. They are facultative anaerobes and are able to ferment carbohydrates. Within this family, microbiologists recognize two distinct categories. The first category is called the coliforms, after its prototypical bacterium species, Escherichia coli. Coliforms are able to ferment lactose completely (i.e., with the production of acid and gas). The second category, noncoliforms, either cannot ferment lactose or can only ferment it incompletely (producing either acid or gas, but not both). The noncoliforms include some notable human pathogens, such as Salmonella spp., Shigella spp., and Yersinia pestis.
E. coli has been perhaps the most studied bacterium since it was first described in 1886 by Theodor Escherich (1857–1911). Many strains of E. coli are in mutualistic relationships with humans. However, some strains produce a potentially deadly toxin called Shiga toxin. Shiga toxin is one of the most potent bacterial toxins identified. Upon entering target cells, Shiga toxin interacts with ribosomes, stopping protein synthesis. Lack of protein synthesis leads to cellular death and hemorrhagic colitis, characterized by inflammation of intestinal tract and bloody diarrhea. In the most severe cases, patients can develop a deadly hemolytic uremic syndrome. Other E. coli strains may cause traveler’s diarrhea, a less severe but very widespread disease.
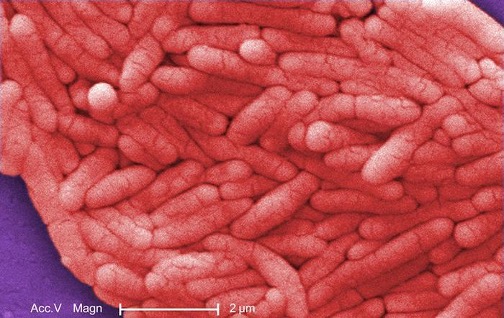
The genus Salmonella, which belongs to the noncoliform group of Enterobacteriaceae, is interesting in that there is still no consensus about how many species it includes. Scientists have reclassified many of the groups they once thought to be species as serotypes (also called serovars), which are strains or variations of the same species of bacteria. Their classification is based on patterns of reactivity by animal antisera against molecules on the surface of the bacterial cells. A number of serotypes of Salmonella can cause salmonellosis, characterized by inflammation of the small and the large intestine, accompanied by fever, vomiting, and diarrhea. The species S. enterobacterica (serovar typhi) causes typhoid fever, with symptoms including fever, abdominal pain, and skin rashes (figure 2.28).
Table 2.9 summarizes the characteristics of important genera of Gammaproteobacteria.
Example Genus | Microscopic Morphology | Unique Characteristics |
---|---|---|
Beggiatoa | Gram-negative bacteria; disc-shaped or cylindrical | Aquatic, live in water with high content of hydrogen disulfide; can cause problems for sewage treatment |
Enterobacter | Gram-negative bacillus | Facultative anaerobe; cause urinary and respiratory tract infections in hospitalized patients; implicated in the pathogenesis of obesity |
Erwinia | Gram-negative bacillus | Plant pathogen causing leaf spots and discoloration; may digest cellulose; prefer relatively low temperatures (25–30 °C) |
Escherichia | Gram-negative bacillus | Facultative anaerobe; inhabit the gastrointestinal tract of warm-blooded animals; some strains are mutualists, producing vitamin K; others, like serotype E. coli O157:H7, are pathogens; E. coli has been a model organism for many studies in genetics and molecular biology |
Hemophilus | Gram-negative bacillus | Pleomorphic, may appear as coccobacillus, aerobe, or facultative anaerobe; grow on blood agar; pathogenic species can cause respiratory infections, chancroid, and other diseases |
Klebsiella | Gram-negative bacillus; appears rounder and thicker than other members of Enterobacteriaceae | Facultative anaerobe, encapsulated, nonmotile; pathogenic species may cause pneumonia, especially in people with alcoholism |
Legionella | Gram-negative bacillus | Fastidious, grow on charcoal-buffered yeast extract; L. pneumophila causes Legionnaires disease |
Methylomonas | Gram-negative bacillus | Use methane as source of carbon and energy |
Proteus | Gram-negative bacillus (pleomorphic) | Common inhabitants of the human gastrointestinal tract; motile; produce urease; opportunistic pathogens; may cause urinary tract infections and sepsis |
Pseudomonas | Gram-negative bacillus | Aerobic; versatile; produce yellow and blue pigments, making them appear green in culture; opportunistic, antibiotic-resistant pathogens may cause wound infections, hospital-acquired infections, and secondary infections in patients with cystic fibrosis |
Serratia | Gram-negative bacillus | Motile; may produce red pigment; opportunistic pathogens responsible for a large number of hospital-acquired infections |
Shigella | Gram-negative bacillus | Nonmotile; dangerously pathogenic; produce Shiga toxin, which can destroy cells of the gastrointestinal tract; can cause dysentery |
Vibrio | Gram-negative, comma- or curved rod-shaped bacteria | Inhabit seawater; flagellated, motile; may produce toxin that causes hypersecretion of water and electrolytes in the gastrointestinal tract; some species may cause serious wound infections |
Yersinia | Gram-negative bacillus | Carried by rodents; human pathogens; Y. pestis causes bubonic plague and pneumonic plague; Y. enterocolitica can be a pathogen causing diarrhea in humans |
Table 2.9: Class Gammaproteobacteria
Deltaproteobacteria
The Deltaproteobacteria is a small class of gram-negative Proteobacteria that includes sulfate-reducing bacteria (SRBs), so named because they use sulfate as the final electron acceptor in the electron transport chain. Few SRBs are pathogenic. However, the SRB Desulfovibrio orale is associated with periodontal disease (disease of the gums).
Deltaproteobacteria also includes the genus Bdellovibrio, species of which are parasites of other gram-negative bacteria. Bdellovibrio invades the cells of the host bacterium, positioning itself in the periplasm, the space between the plasma membrane and the cell wall, feeding on the host’s proteins and polysaccharides. The infection is lethal for the host cells.
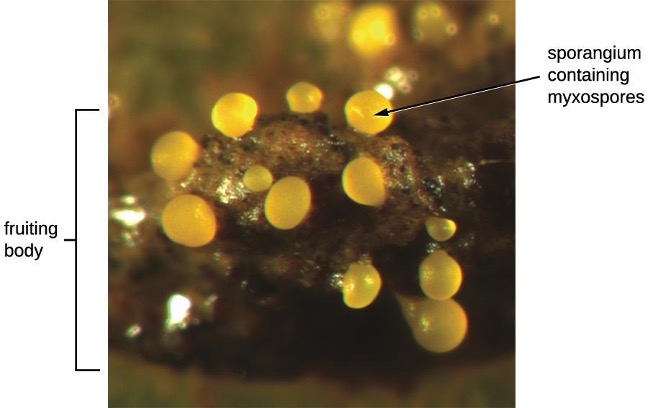
Another type of Deltaproteobacteria, myxobacteria, lives in the soil, scavenging inorganic compounds. Motile and highly social, they interact with other bacteria within and outside their own group. They can form multicellular, macroscopic fruiting bodies (figure 2.29), structures that are still being studied by biologists and bacterial ecologists.[7] These bacteria can also form metabolically inactive myxospores.
Table 2.10 summarizes the characteristics of several important genera of Deltaproteobacteria.
Genus | Microscopic Morphology | Unique Characteristics |
---|---|---|
Bdellovibrio | Gram-negative, comma-shaped rod | Obligate aerobes; motile; parasitic (infecting other bacteria) |
Desulfovibrio (formerly Desufuromonas) | Gram-negative, comma-shaped rod | Reduce sulfur; can be used for removal of toxic and radioactive waste |
Myxobacterium | Gram-negative, coccoid bacteria forming colonies (swarms) | Live in soil; can move by gliding; used as a model organism for studies of intercellular communication (signaling) |
Table 2.10: Class Deltaproteobacteria
Epsilonproteobacteria
The smallest class of Proteobacteria is Epsilonproteobacteria, which are gram-negative microaerophilic bacteria (meaning they only require small amounts of oxygen in their environment). Two clinically relevant genera of Epsilonproteobacteria are Campylobacter and Helicobacter, both of which include human pathogens. Campylobacter can cause food poisoning that manifests as severe enteritis (inflammation in the small intestine). This condition, caused by the species C. jejuni, is rather common in developed countries, usually because of eating contaminated poultry products. Chickens often harbor C. jejuni in their gastrointestinal tract and feces, and their meat can become contaminated during processing.
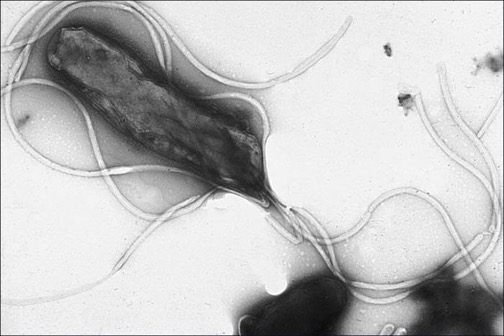
Within the genus Helicobacter, the helical, flagellated bacterium H. pylori has been identified as a beneficial member of the stomach microbiota, but it is also the most common cause of chronic gastritis and ulcers of the stomach and duodenum (figure 2.30). Studies have also shown that H. pylori is linked to stomach cancer.[8] H. pylori is somewhat unusual in its ability to survive in the highly acidic environment of the stomach. It produces urease and other enzymes that modify its environment to make it less acidic.
Table 2.11 summarizes the characteristics of the most clinically relevant genera of Epsilonproteobacteria.
Example Genus | Microscopic Morphology | Unique Characteristics |
---|---|---|
Campylobacter | Gram-negative, spiral-shaped rod | Aerobic (microaerophilic); often infects chickens; may infect humans via undercooked meat, causing severe enteritis |
Helicobacter | Gram-negative, spiral-shaped rod | Aerobic (microaerophilic) bacterium; can damage the inner lining of the stomach, causing chronic gastritis, peptic ulcers, and stomach cancer |
Table 2.11: Class Epsilonproteobacteria
2.5 Nonproteobacteria Gram-Negative Bacteria and Phototrophic Bacteria
The majority of the gram-negative bacteria belong to the phylum Proteobacteria. Those that do not are called the nonproteobacteria. In this section, we will describe four classes of gram-negative nonproteobacteria: Chlamydia, the spirochetes, the CFB group, and the Planctomycetes. A diverse group of phototrophic bacteria that includes Proteobacteria and nonproteobacteria will be discussed at the end of this section.
Chlamydia
C. trachomatis is a human pathogen that causes trachoma, a disease of the eyes, often leading to blindness. C. trachomatis also causes the sexually transmitted disease lymphogranuloma venereum (LGV). This disease is often mildly symptomatic, manifesting as regional lymph node swelling, or it may be asymptomatic, but it is extremely contagious and is common on college campuses.
Members of the genus Chlamydia are gram-negative, obligate intracellular pathogens that are extremely resistant to the cellular defenses, giving them the ability to spread from host to host rapidly via elementary bodies. The metabolically and reproductively inactive elementary bodies are an endospore-like form of intracellular bacteria that enter an epithelial cell, where they become active. Figure 2.31 illustrates the life cycle of Chlamydia.
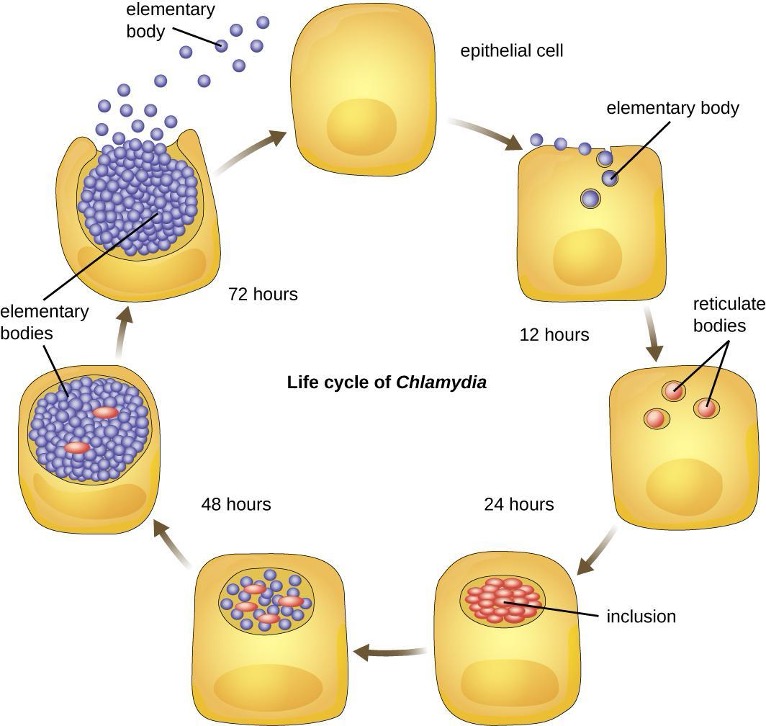
Spirochetes
Spirochetes are characterized by their long (up to 250 μm), spiral-shaped bodies. Most spirochetes are also very thin, which makes it difficult to examine gram-stained preparations under a conventional brightfield microscope. Darkfield fluorescent microscopy is typically used instead. Spirochetes are also difficult or even impossible to culture. They are highly motile, using their axial filament to propel themselves. The axial filament is similar to a flagellum, but it wraps around the cell and runs inside the cell body of a spirochete in the periplasmic space between the outer membrane and the plasma membrane (figure 2.32).
Several genera of spirochetes include human pathogens. For example, the genus Treponema includes a species T. pallidum, which is further classified into four subspecies: T. pallidum pallidum, T. pallidum pertenue, T. pallidum carateum, and T. pallidum endemicum. The subspecies T. pallidum pallidum causes the sexually transmitted infection known as syphilis, the third most prevalent sexually transmitted bacterial infection in the United States, after chlamydia and gonorrhea. The other subspecies of T. pallidum cause tropical infectious diseases of the skin, bones, and joints.
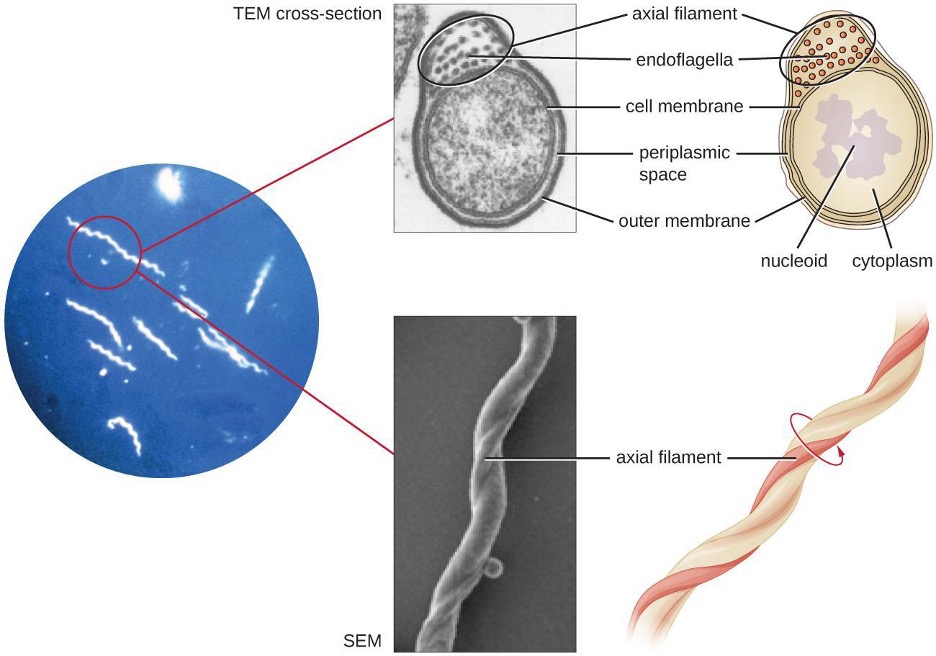
Another genus of spirochete, Borrelia, contains a number of pathogenic species. B. burgdorferi causes Lyme disease, which is transmitted by several genera of ticks (notably Ixodes and Amblyomma) and often produces a bull’s eye rash, fever, fatigue, and, sometimes, debilitating arthritis. B. recurrens causes a condition known as relapsing fever.
Cytophaga, Fusobacterium, and Bacteroides
The gram-negative nonproteobacteria of the genera Cytophaga, Fusobacterium, and Bacteroides are classified together as a phylum and called the CFB group. Although they are phylogenetically diverse, bacteria of the CFB group share some similarities in the sequence of nucleotides in their DNA. They are rod-shaped bacteria adapted to anaerobic environments, such as the tissue of the gums, gut, and rumen of ruminating animals. CFB bacteria are avid fermenters, able to process cellulose in rumen, thus enabling ruminant animals to obtain carbon and energy from grazing.
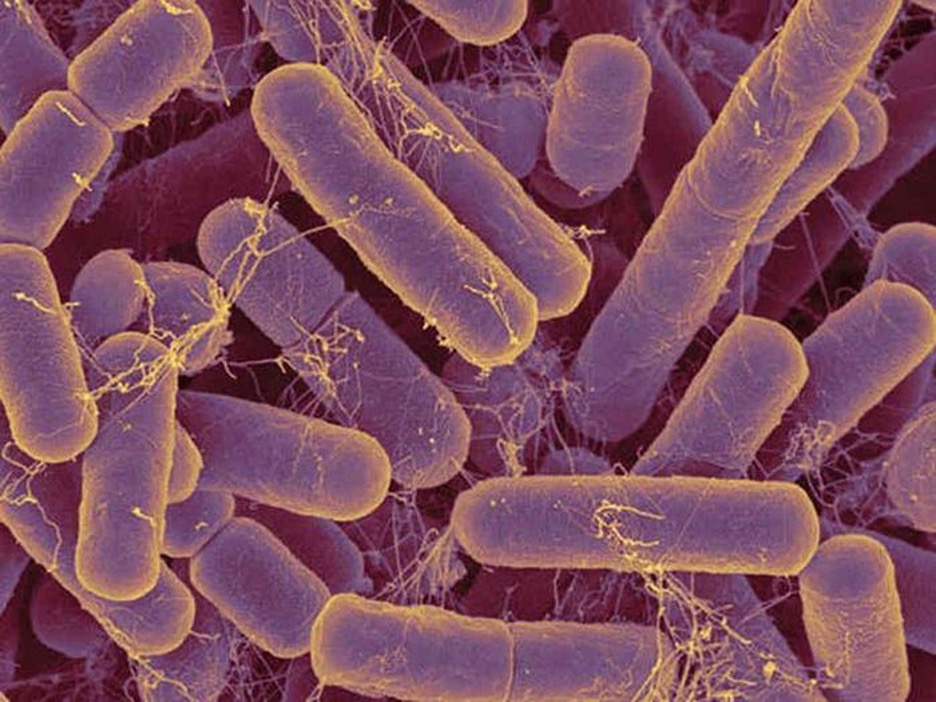
Cytophaga are motile aquatic bacteria that glide. Fusobacteria inhabit the human mouth and may cause severe infectious diseases. The largest genus of the CFB group is Bacteroides, which includes dozens of species that are prevalent inhabitants of the human large intestine, making up about 30% of the entire gut microbiome (figure 2.33). One gram of human feces contains up to 100 billion Bacteroides cells. Most Bacteroides are mutualistic. They benefit from nutrients they find in the gut, and humans benefit from their ability to prevent pathogens from colonizing the large intestine. Indeed, when populations of Bacteroides are reduced in the gut—as often occurs when a patient takes antibiotics—the gut becomes a more favorable environment for pathogenic bacteria and fungi, which can cause secondary infections.
Only a few species of Bacteroides are pathogenic. B. melaninogenicus, for example, can cause wound infections in patients with weakened immune systems.
Planctomycetes
The Planctomycetes are found in aquatic environments, inhabiting freshwater, saltwater, and brackish water. Planctomycetes are unusual in that they reproduce by budding, meaning that instead of one maternal cell splitting into two equal daughter cells in the process of binary fission, the mother cell forms a bud that detaches and lives as an independent cell. These so-called swarmer cells are motile and not attached to a surface. However, they will soon differentiate into sessile (immobile) cells with an appendage called a holdfast that allows them to attach to surfaces in the water (figure 2.34). Only the sessile cells are able to reproduce.
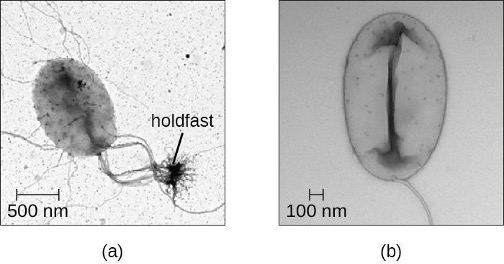
Table 2.12 summarizes the characteristics of some of the most clinically relevant genera of nonproteobacteria.
Example Genus | Microscopic Morphology | Unique Characteristics |
---|---|---|
Chlamydia | Gram-negative, coccoid or ovoid bacterium | Obligatory intracellular bacteria; some cause chlamydia, trachoma, and pneumonia |
Bacteroides | Gram-negative bacillus | Obligate anaerobic bacteria; abundant in the human gastrointestinal tract; usually mutualistic, although some species are opportunistic pathogens |
Cytophaga | Gram-negative bacillus | Motile by gliding; live in soil or water; decompose cellulose; may cause disease in fish |
Fusobacterium | Gram-negative bacillus with pointed ends | Anaerobic; form biofilms; some species cause disease in humans (periodontitis, ulcers) |
Leptospira | Spiral-shaped bacterium (spirochetes); gram negative-like (better viewed by darkfield microscopy); very thin | Aerobic, abundant in shallow water reservoirs; infect rodents and domestic animals; can be transmitted to humans by infected animals’ urine; may cause severe disease |
Borrelia | Gram-negative-like spirochete; very thin; better viewed by darkfield microscopy | B. burgdorferi causes Lyme disease and B. recurrens causes relapsing fever |
Treponema | Gram-negative-like spirochete; very thin; better viewed by darkfield microscopy | Motile; do not grow in culture; T. pallidum (subspecies T. pallidum pallidum) causes syphilis |
Table 2.12: Class nonproteobacteria
Phototrophic Bacteria
The phototrophic bacteria are a large and diverse category of bacteria that do not represent a taxon but, rather, a group of bacteria that use sunlight as their primary source of energy. This group contains both Proteobacteria and nonproteobacteria. They use solar energy to synthesize ATP through photosynthesis. When they produce oxygen, they perform oxygenic photosynthesis. When they do not produce oxygen, they perform anoxygenic photosynthesis. With the exception of some cyanobacteria, the majority of phototrophic bacteria perform anoxygenic photosynthesis.
One large group of phototrophic bacteria includes the purple or green bacteria that perform photosynthesis with the help of bacteriochlorophylls, which are green, purple, or blue pigments similar to chlorophyll in plants. Some of these bacteria have a varying amount of red or orange pigments called carotenoids. Their color varies from orange to red to purple to green (figure 2.35), and they are able to absorb light of various wavelengths. Traditionally, these bacteria are classified into sulfur and nonsulfur bacteria; they are further differentiated by color.
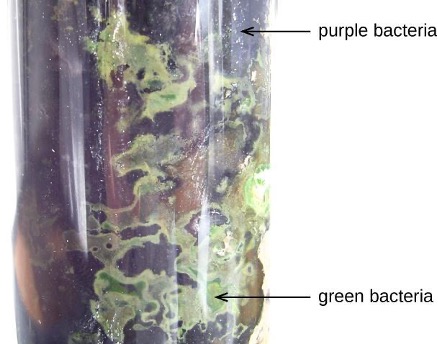
The sulfur bacteria perform anoxygenic photosynthesis, using sulfites as electron donors as well as releasing free elemental sulfur. Nonsulfur bacteria use organic substrates, such as succinate and malate, as donors of electrons.
The purple sulfur bacteria oxidize hydrogen sulfide into elemental sulfur and sulfuric acid. They get their purple color from the pigments bacteriochlorophylls and carotenoids. Bacteria of the genus Chromatium are purple sulfur Gammaproteobacteria. These microorganisms are strict anaerobes and live in water. They use carbon dioxide as their only source of carbon, but their survival and growth are possible only in the presence of sulfites, which they use as electron donors. Chromatium has been used as a model for studies of bacterial photosynthesis since the 1950s.[9]
The green sulfur bacteria use sulfide for oxidation and produce large amounts of green bacteriochlorophyll. The genus Chlorobium is a green sulfur bacterium that is implicated in climate change because it produces methane, a greenhouse gas. These bacteria use at least four types of chlorophyll for photosynthesis. The most prevalent of these, bacteriochlorophyll, is stored in special vesicle-like organelles called chlorosomes.
Purple nonsulfur bacteria are similar to purple sulfur bacteria, except that they use hydrogen rather than hydrogen sulfide for oxidation. Among the purple nonsulfur bacteria is the genus Rhodospirillum. These microorganisms are facultative anaerobes, which are actually pink rather than purple, and can metabolize (“fix”) nitrogen. They may be valuable in the field of biotechnology because of their potential ability to produce biological plastic and hydrogen fuel.[10]
The green nonsulfur bacteria are similar to green sulfur bacteria except for their use of substrates other than sulfides for oxidation. Chloroflexus is an example of a green nonsulfur bacterium. It often has an orange color when it grows in the dark, but it becomes green when it grows in sunlight. It stores bacteriochlorophyll in chlorosomes, similar to Chlorobium, and performs anoxygenic photosynthesis, using organic sulfites (low concentrations) or molecular hydrogen as electron donors, so it can survive in the dark if oxygen is available. Chloroflexus does not have flagella but can glide, like Cytophaga. It grows at a wide range of temperatures, from 35 °C to 70 °C, thus can be thermophilic.
Another large, diverse group of phototrophic bacteria compose the phylum Cyanobacteria; they get their blue-green color from the chlorophyll contained in their cells (figure 2.36). Species of this group perform oxygenic photosynthesis, producing megatons of gaseous oxygen. Scientists hypothesize that cyanobacteria played a critical role in the change of our planet’s anoxic atmosphere 1–2 billion years ago to the oxygen-rich environment we have today.[11]
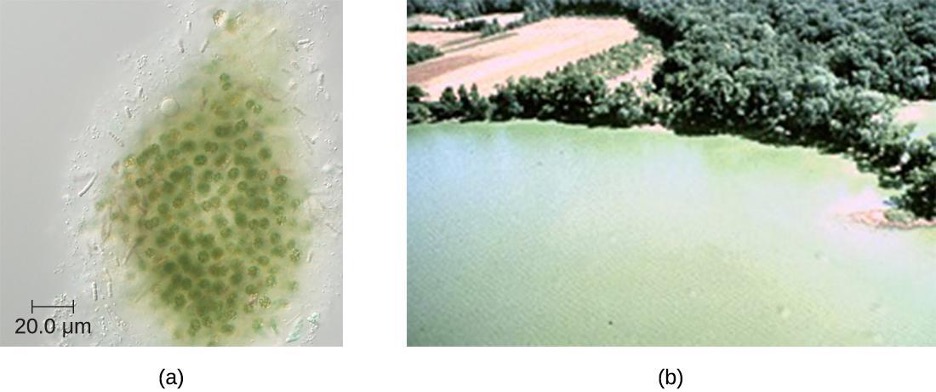
Cyanobacteria have other remarkable properties. Amazingly adaptable, they thrive in many habitats, including marine and freshwater environments, soil, and even rocks. They can live at a wide range of temperatures, even in the extreme temperatures of the Antarctic. They can live as unicellular organisms or in colonies, and they can be filamentous, forming sheaths or biofilms. Many of them fix nitrogen, converting molecular nitrogen into nitrites and nitrates that other bacteria, plants, and animals can use. The reactions of nitrogen fixation occur in specialized cells called heterocysts.
Photosynthesis in Cyanobacteria is oxygenic, using the same type of chlorophyll found in plants and algae as the primary photosynthetic pigment. Cyanobacteria also use phycocyanin and cyanophycin, two secondary photosynthetic pigments that give them their characteristic blue color. They are located in special organelles called phycobilisomes and in folds of the cellular membrane called thylakoids, which are remarkably similar to the photosynthetic apparatus of plants. Scientists hypothesize that plants originated from endosymbiosis of ancestral eukaryotic cells and ancestral photosynthetic bacteria.[12] Cyanobacteria are also an interesting object of research in biochemistry,[13] with studies investigating their potential as biosorbents[14] and products of human nutrition.[15]
Unfortunately, cyanobacteria can sometimes have a negative impact on human health. Genera such as Microcystis can form harmful cyanobacterial blooms, forming dense mats on bodies of water and producing large quantities of toxins that can harm wildlife and humans. These toxins have been implicated in tumors of the liver and diseases of the nervous system in animals and humans.[16]
Table 2.13 summarizes the characteristics of important phototrophic bacteria.
Phylum | Class | Example Genus or Species | Common Name | Oxygenic or Anoxygenic | Sulfur Deposition |
---|---|---|---|---|---|
Cyanobacteria | Cyanophyceae | Microcystis aeruginosa | Blue-green bacteria | Oxygenic | None |
Chlorobi | Chlorobia | Chlorobium | Green sulfur bacteria | Anoxygenic | Outside the cell |
Chloroflexi (Division) | Chloroflexi | Chloroflexus | Green nonsulfur bacteria | Anoxygenic | None |
Proteobacteria | Alphaproteobacteria | Rhodospirillum | Purple nonsulfur bacteria | Anoxygenic | None |
Betaproteobacteria | Rhodocyclus | Purple nonsulfur bacteria | Anoxygenic | None | |
Gammaproteobacteria | Chromatium | Purple sulfur bacteria | Anoxygenic | Inside the cell |
Table 2.13: Phototrophic bacteria
2.6 Gram-Positive Bacteria
Prokaryotes are identified as gram-positive if they have a multiple layer matrix of peptidoglycan forming the cell wall. Advances in nucleic acid biochemistry have revealed additional characteristics that can be used to classify gram-positive prokaryotes, namely the guanine to cytosine ratios (G+C) in DNA and the composition of 16S rRNA subunits. Microbiologists currently recognize two distinct groups of gram-positive, or weakly staining gram-positive, prokaryotes. The class Actinobacteria comprises the high G+C gram-positive bacteria, which have more than 50% guanine and cytosine nucleotides in their DNA. The class Bacilli comprises low G+C gram-positive bacteria, which have less than 50% of guanine and cytosine nucleotides in their DNA.
Actinobacteria: High G+C Gram-Positive Bacteria
The name Actinobacteria comes from the Greek words for rays and small rod, but Actinobacteria are very diverse. Their microscopic appearance can range from thin filamentous branching rods to coccobacilli. Most Actinobacteria live in the soil, but some are aquatic. The vast majority are aerobic. One distinctive feature of this group is the presence of several different peptidoglycans in the cell wall.
Actinomyces spp. play an important role in soil ecology, and some species are human pathogens. A number of Actinomyces spp. inhabit the human mouth and are opportunistic pathogens, causing infectious diseases like periodontitis (inflammation of the gums) and oral abscesses. The species A. israelii is an anaerobe notorious for causing endocarditis (inflammation of the inner lining of the heart) (figure 2.37).
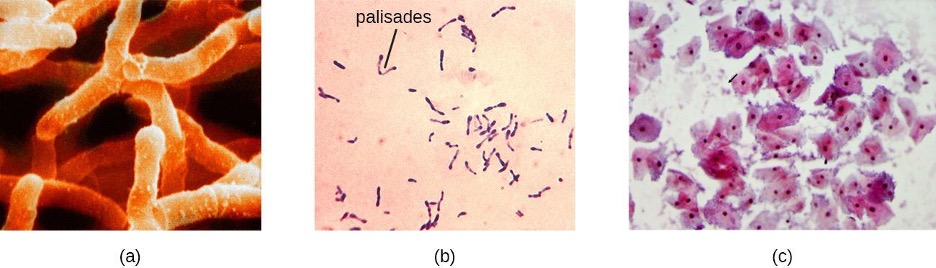
The genus Mycobacterium is represented by bacilli covered with a mycolic acid coat. This waxy coat protects the bacteria from some antibiotics, prevents them from drying out, and blocks penetration by Gram stain reagents (see section 2.2). Because of this, a special acid-fast staining procedure is used to visualize these bacteria. The genus Mycobacterium is an important cause of a diverse group of infectious diseases. M. tuberculosis is the causative agent of tuberculosis, a disease that primarily impacts the lungs but can infect other parts of the body as well. It has been estimated that one-third of the world’s population has been infected with M. tuberculosis and millions of new infections occur each year. Treatment of M. tuberculosis is challenging and requires patients to take a combination of drugs for an extended time. Complicating treatment even further is the development and spread of multidrug-resistant strains of this pathogen.
Another pathogenic species, M. leprae, is the cause of Hansen’s disease (leprosy), a chronic disease that impacts peripheral nerves and the integrity of the skin and mucosal surface of the respiratory tract. Loss of pain sensation and the presence of skin lesions increase susceptibility to secondary injuries and infections with other pathogens.
Bacteria in the genus Corynebacterium contain diaminopimelic acid in their cell walls, and microscopically often form palisades, or pairs of rod-shaped cells resembling the letter V. Cells may contain metachromatic granules, intracellular storage of inorganic phosphates that are useful for identification of Corynebacterium. The vast majority of Corynebacterium spp. are nonpathogenic; however, C. diphtheria is the causative agent of diphtheria, a disease that can be fatal, especially in children (figure 2.37). C. diphtheria produces a toxin that forms a pseudomembrane in the patient’s throat, causing swelling, difficulty breathing, and other symptoms that can become serious if untreated.
The genus Bifidobacterium consists of filamentous anaerobes, many of which are commonly found in the gastrointestinal tract, vagina, and mouth. In fact, Bifidobacterium spp. constitute a substantial part of the human gut microbiota and are frequently used as probiotics and in yogurt production.
The genus Gardnerella, contains only one species, G. vaginalis. This species is defined as gram-variable because its small coccobacilli do not show consistent results when Gram stained (figure 2.37). Based on its genome, it is placed into the high G+C gram-positive group. G. vaginalis can cause bacterial vaginosis in women; symptoms are typically mild or even undetectable, but can lead to complications during pregnancy.
Table 2.14 summarizes the characteristics of some important genera of Actinobacteria.
Example Genus | Microscopic Morphology | Unique Characteristics |
---|---|---|
Actinomyces | Gram-positive bacillus; in colonies, shows fungus-like threads (hyphae) | Facultative anaerobes; in soil, decompose organic matter; in the human mouth, may cause gum disease |
Arthrobacter | Gram-positive bacillus (at the exponential stage of growth) or coccus (in stationary phase) | Obligate aerobes; divide by “snapping,” forming V-like pairs of daughter cells; degrade phenol, can be used in bioremediation |
Bifidobacterium | Gram-positive, filamentous actinobacterium | Anaerobes commonly found in human gut microbiota |
Corynebacterium | Gram-positive bacillus | Aerobes or facultative anaerobes; form palisades; grow slowly; require enriched media in culture; C. diphtheriae causes diphtheria |
Frankia | Gram-positive, fungus-like (filamentous) bacillus | Nitrogen-fixing bacteria; live in symbiosis with legumes |
Gardnerella | Gram-variable coccobacillus | Colonize the human vagina, may alter the microbial ecology, thus leading to vaginosis |
Micrococcus | Gram-positive coccus, form microscopic clusters | Ubiquitous in the environment and on the human skin; oxidase-positive (as opposed to morphologically similar S. aureus); some are opportunistic pathogens |
Mycobacterium | Gram-positive, acid-fast bacillus | Slow growing, aerobic, resistant to drying and phagocytosis; covered with a waxy coat made of mycolic acid; M. tuberculosis causes tuberculosis; M. leprae causes leprosy |
Nocardia | Weakly gram-positive bacillus; forms acid-fast branches | May colonize the human gingiva; may cause severe pneumonia and inflammation of the skin |
Propionibacterium | Gram-positive bacillus | Aerotolerant anaerobe; slow-growing; P. acnes reproduces in the human sebaceous glands and may cause or contribute to acne |
Rhodococcus | Gram-positive bacillus | Strict aerobe; used in industry for biodegradation of pollutants; R. fascians is a plant pathogen, and R. equi causes pneumonia in foals |
Streptomyces | Gram-positive, fungus-like (filamentous) bacillus | Very diverse genus (>500 species); aerobic, spore-forming bacteria; scavengers, decomposers found in soil (give the soil its earthy odor); used in pharmaceutical industry as antibiotic producers (more than two-thirds of clinically useful antibiotics) |
Table 2.14: Actinobacteria: High G+C gram-positive
Low G+C Gram-positive Bacteria
The low G+C gram-positive bacteria have less than 50% guanine and cytosine in their DNA, and this group of bacteria includes a number of genera of bacteria that are pathogenic.
Clostridia
One large and diverse class of low G+C gram-positive bacteria is Clostridia. The best studied genus of this class is Clostridium. These rod-shaped bacteria are generally obligate anaerobes that produce endospores and can be found in anaerobic habitats like soil and aquatic sediments rich in organic nutrients. The endospores may survive for many years.
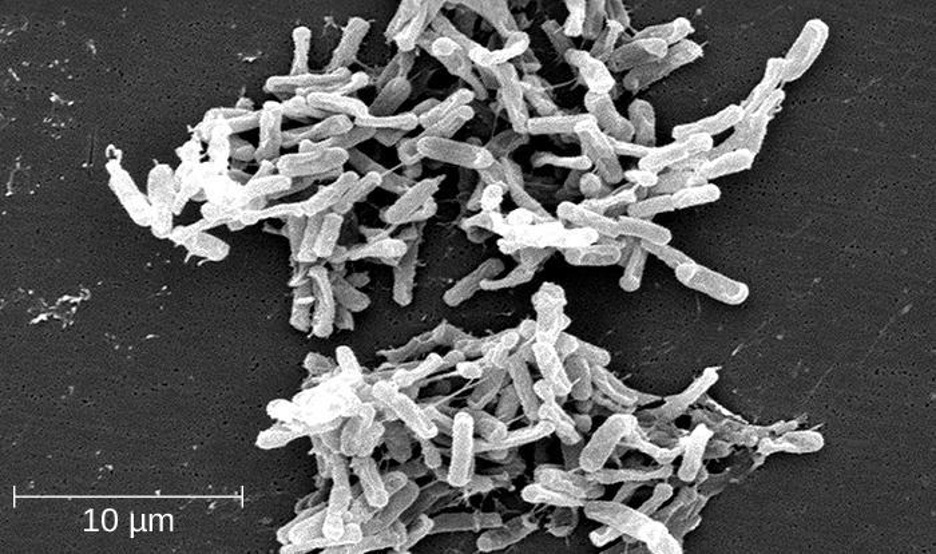
Clostridium spp. produce more kinds of protein toxins than any other bacterial genus, and several species are human pathogens. C. perfringens is the third most common cause of food poisoning in the United States and is the causative agent of an even more serious disease called gas gangrene. Gas gangrene occurs when C. perfringens endospores enter a wound and germinate, becoming viable bacterial cells and producing a toxin that can cause the necrosis (death) of tissue. C. tetani, which causes tetanus, produces a neurotoxin that is able to enter neurons, travel to regions of the central nervous system where it blocks the inhibition of nerve impulses involved in muscle contractions, and cause a life-threatening spastic paralysis. C. botulinum produces botulinum neurotoxin, the most lethal biological toxin known. Botulinum toxin is responsible for rare but frequently fatal cases of botulism. The toxin blocks the release of acetylcholine in neuromuscular junctions, causing flaccid paralysis. In very small concentrations, botulinum toxin has been used to treat muscle pathologies in humans and in a cosmetic procedure to eliminate wrinkles. C. difficile is a common source of hospital-acquired infections (figure 2.38) that can result in serious and even fatal cases of colitis (inflammation of the large intestine). Infections often occur in patients who are immunosuppressed or undergoing antibiotic therapy that alters the normal microbiota of the gastrointestinal tract.
Lactobacillales
The order Lactobacillales comprises low G+C gram-positive bacteria that include both bacilli and cocci in the genera Lactobacillus, Leuconostoc, Enterococcus, and Streptococcus. Bacteria of the latter three genera typically are spherical or ovoid and often form chains.
Streptococcus, the name of which comes from the Greek word for twisted chain, is responsible for many types of infectious diseases in humans. Species from this genus, often referred to as streptococci, are usually classified by serotypes called Lancefield groups, and by their ability to lyse red blood cells when grown on blood agar.
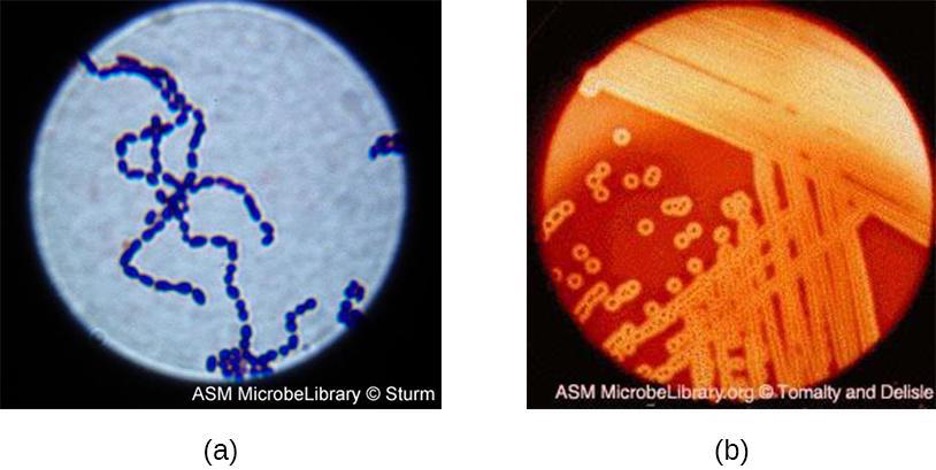
S. pyogenes belongs to the Lancefield group A, β-hemolytic Streptococcus. This species is considered a pyogenic pathogen because of the associated pus production observed with infections it causes (figure 2.39). S. pyogenes is the most common cause of bacterial pharyngitis (strep throat); it is also an important cause of various skin infections that can be relatively mild (e.g., impetigo) or life threatening (e.g., necrotizing fasciitis, also known as flesh eating disease).
The nonpyogenic (i.e., not associated with pus production) streptococci are a group of streptococcal species that are not a taxon but are grouped together because they inhabit the human mouth. The nonpyogenic streptococci do not belong to any of the Lancefield groups. Most are commensals, but a few, such as S. mutans, are implicated in the development of dental caries.
S. pneumoniae (commonly referred to as pneumococcus), is a Streptococcus species that also does not belong to any Lancefield group. S. pneumoniae cells appear microscopically as diplococci, pairs of cells, rather than the long chains typical of most streptococci. Scientists have known since the 19th century that S. pneumoniae causes pneumonia and other respiratory infections. However, this bacterium can also cause a wide range of other diseases, including meningitis, septicemia, osteomyelitis, and endocarditis, especially in newborns, the elderly, and patients with immunodeficiency.
Bacilli
The name of the class Bacilli suggests that it is made up of bacteria that are bacillus in shape, but it is a morphologically diverse class that includes bacillus-shaped and cocccus-shaped genera. Among the many genera in this class are two that are very important clinically: Bacillus and Staphylococcus.
Bacteria in the genus Bacillus are bacillus in shape and can produce endospores. They include aerobes or facultative anaerobes. A number of Bacillus spp. are used in various industries, including the production of antibiotics (e.g., barnase), enzymes (e.g., alpha-amylase, BamH1 restriction endonuclease), and detergents (e.g., subtilisin).
Two notable pathogens belong to the Bacillus genus. B. anthracis is the pathogen that causes anthrax, a severe disease that affects wild and domesticated animals and can spread from infected animals to humans. Anthrax manifests in humans as charcoal-black ulcers on the skin, severe enterocolitis, pneumonia, and brain damage due to swelling. If untreated, anthrax is lethal. B. cereus, a closely related species, is a pathogen that may cause food poisoning. It is a rod-shaped species that forms chains. Colonies appear milky white with irregular shapes when cultured on blood agar (figure 2.40). One other important species is B. thuringiensis. This bacterium produces a number of substances used as insecticides because they are toxic for insects.
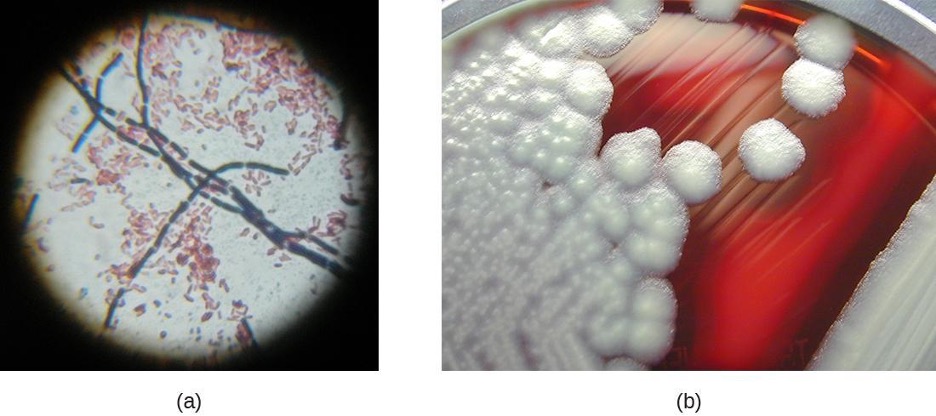
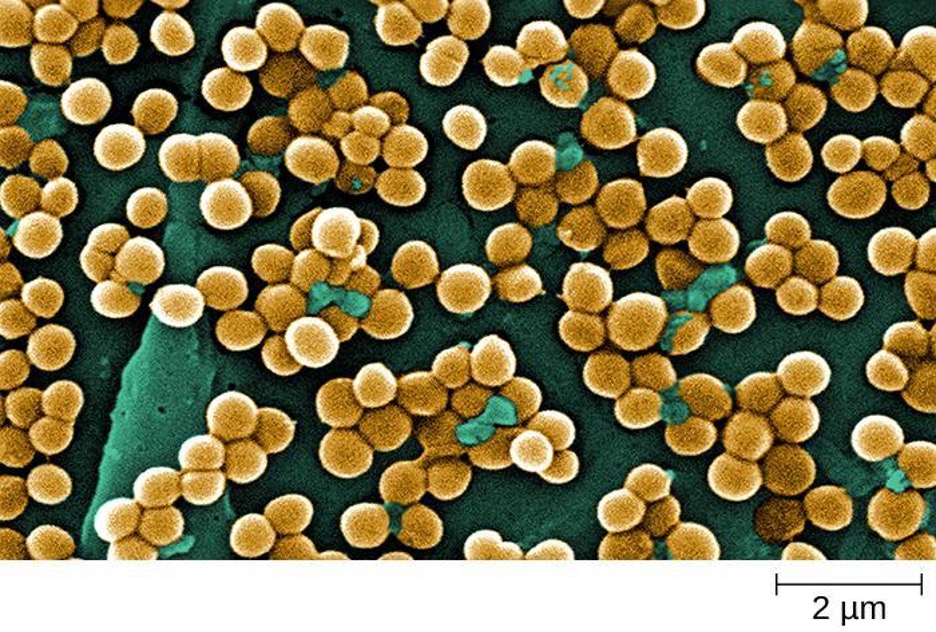
The genus Staphylococcus also belongs to the class Bacilli, even though its shape is coccus rather than a bacillus. The name Staphylococcus comes from a Greek word for bunches of grapes, which describes their microscopic appearance in cultures (figure 2.41). Staphylococcus spp. are facultative anaerobic, halophilic, and nonmotile. The two best-studied species of this genus are S. epidermidis and S. aureus.
S. epidermidis, whose main habitat is the human skin, is thought to be nonpathogenic for humans with healthy immune systems, but in patients with immunodeficiency, it may cause infections in skin wounds and prostheses (e.g., artificial joints, heart valves). S. epidermidis is also an important cause of infections associated with intravenous catheters. This makes it a dangerous pathogen in hospital settings, where many patients may be immunocompromised.
Strains of S. aureus cause a wide variety of infections in humans, including skin infections that produce boils, carbuncles, cellulitis, or impetigo. Certain strains of S. aureus produce a substance called enterotoxin, which can cause severe enteritis, often called staph food poisoning. Some strains of S. aureus produce the toxin responsible for toxic shock syndrome, which can result in cardiovascular collapse and death.
Many strains of S. aureus have developed resistance to antibiotics. Some antibiotic-resistant strains are designated as methicillin-resistant S. aureus (MRSA) and vancomycin-resistant S. aureus (VRSA). These strains are some of the most difficult to treat because they exhibit resistance to nearly all available antibiotics, not just methicillin and vancomycin. Because they are difficult to treat with antibiotics, infections can be lethal. MRSA and VRSA are also contagious, posing a serious threat in hospitals, nursing homes, dialysis facilities, and other places where there are large populations of elderly, bedridden, and/or immunocompromised patients.
Mycoplasmas
Although Mycoplasma spp. do not possess a cell wall and, therefore, are not stained by Gram-stain reagents, this genus is still included with the low G+C gram-positive bacteria. The genus Mycoplasma includes more than 100 species, which share several unique characteristics. They are very small cells, some with a diameter of about 0.2 μm, which is smaller than some large viruses. They have no cell walls and, therefore, are pleomorphic, meaning that they may take on a variety of shapes and can even resemble very small animal cells. Because they lack a characteristic shape, they can be difficult to identify. One species, M. pneumoniae, causes the mild form of pneumonia known as “walking pneumonia” or “atypical pneumonia.” This form of pneumonia is typically less severe than forms caused by other bacteria or viruses.
Table 2.15 summarizes the characteristics of notable genera low G+C Gram-positive bacteria.
Example Genus | Microscopic Morphology | Unique Characteristics |
---|---|---|
Bacillus | Large, gram-positive bacillus | Aerobes or facultative anaerobes; form endospores; B. anthracis causes anthrax in cattle and humans, B. cereus may cause food poisoning |
Clostridium | Gram-positive bacillus | Strict anaerobes; form endospores; all known species are pathogenic, causing tetanus, gas gangrene, botulism, and colitis |
Enterococcus | Gram-positive coccus; forms microscopic pairs in culture (resembling Streptococcus pneumoniae) | Anaerobic aerotolerant bacteria, abundant in the human gut, may cause urinary tract and other infections in the nosocomial environment |
Lactobacillus | Gram-positive bacillus | Facultative anaerobes; ferment sugars into lactic acid; part of the vaginal microbiota; used as probiotics |
Leuconostoc | Gram-positive coccus; may form microscopic chains in culture | Fermenter, used in food industry to produce sauerkraut and kefir |
Mycoplasma | The smallest bacteria; appear pleomorphic under electron microscope | Have no cell wall; classified as low G+C Gram-positive bacteria because of their genome; M. pneumoniae causes “walking” pneumonia |
Staphylococcus | Gram-positive coccus; forms microscopic clusters in culture that resemble bunches of grapes | Tolerate high salt concentration; facultative anaerobes; produce catalase; S. aureus can also produce coagulase and toxins responsible for local (skin) and generalized infections |
Streptococcus | Gram-positive coccus; forms chains or pairs in culture | Diverse genus; classified into groups based on sharing certain antigens; some species cause hemolysis and may produce toxins responsible for human local (throat) and generalized disease |
Ureaplasma | Similar to Mycoplasma | Part of the human vaginal and lower urinary tract microbiota; may cause inflammation, sometimes leading to internal scarring and infertility |
Table 2.15: Bacilli: Low G+C gram-positive bacteria
2.7 Unicellular Eukaryotic Parasites
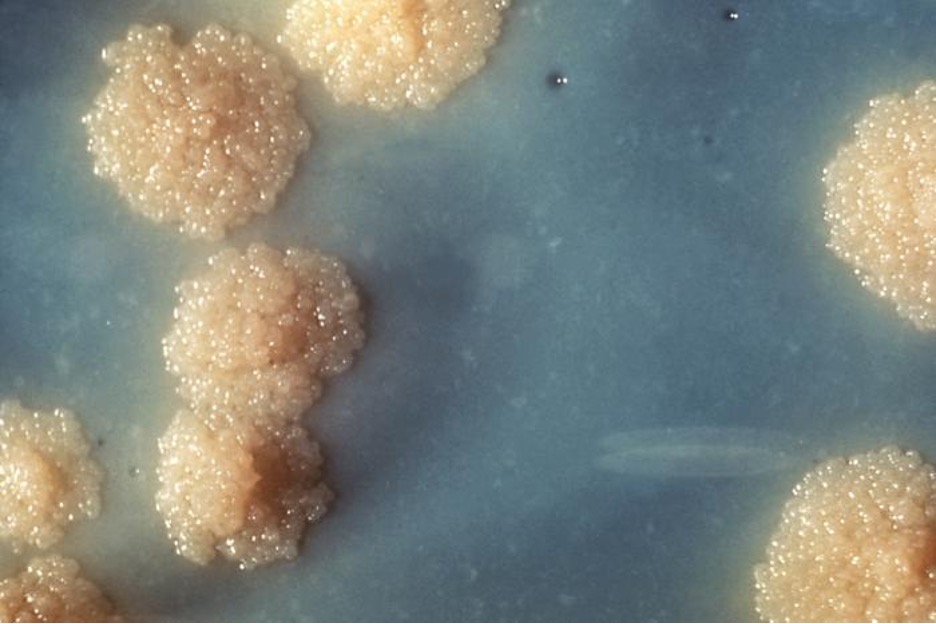
Eukaryotic microbes are an extraordinarily diverse group, including species with a wide range of life cycles, morphological specializations, and nutritional needs (table 2.16). Although more diseases are caused by viruses and bacteria than by microscopic eukaryotes, these eukaryotes are responsible for some diseases of great public health importance. The protist parasite Giardia causes a diarrheal illness (giardiasis) that is easily transmitted through contaminated water supplies. In the United States, Giardia is the most common human intestinal parasite (figure 2.44). Even in developed countries, these worms are notable parasites affecting humans and domestic animals. There are fewer fungal pathogens, but these are also relevant causes of illness, as well. At the same time,, fungi have been important in producing antimicrobial substances such as penicillin.
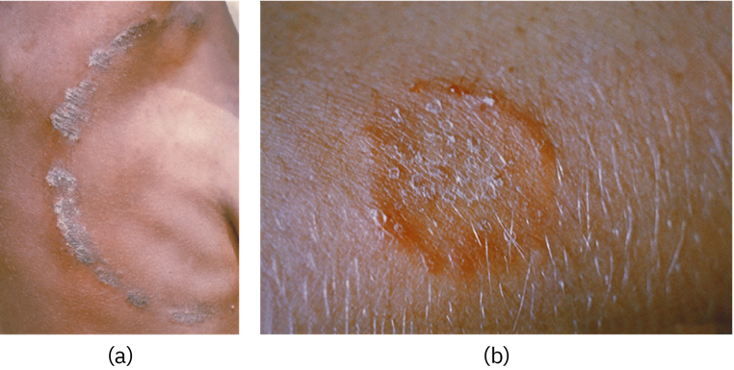
In this section, we will examine characteristics of protists, worms, and fungi while considering their roles in causing disease.
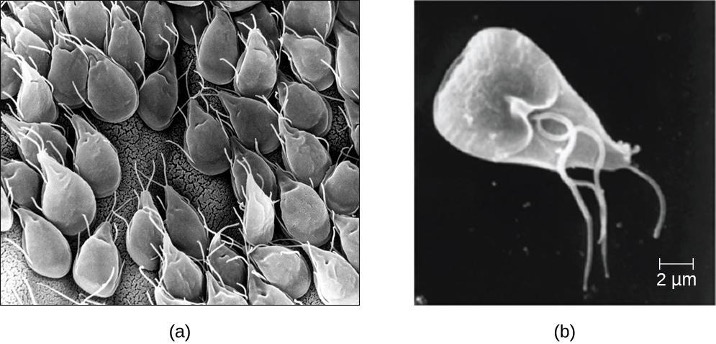
Characteristics of Protists
Protozoans inhabit a wide variety of habitats, both aquatic and terrestrial. Many are free-living, while others are parasitic, carrying out a life cycle within a host or hosts and potentially causing illness. There are also beneficial symbionts that provide metabolic services to their hosts. During the feeding and growth part of their life cycle, they are called trophozoites; these feed on small particulate food sources such as bacteria. While some types of protozoa exist exclusively in the trophozoite form, others can develop from trophozoite to an encapsulated cyst stage when environmental conditions are too harsh for the trophozoite. A cyst is a cell with a protective wall, and the process by which a trophozoite becomes a cyst is called encystment. When conditions become more favorable, these cysts are triggered by environmental cues to become active again through excystment.
One protozoan genus capable of encystment is Eimeria, which includes some human and animal pathogens. Figure 2.45 illustrates the life cycle of Eimeria.
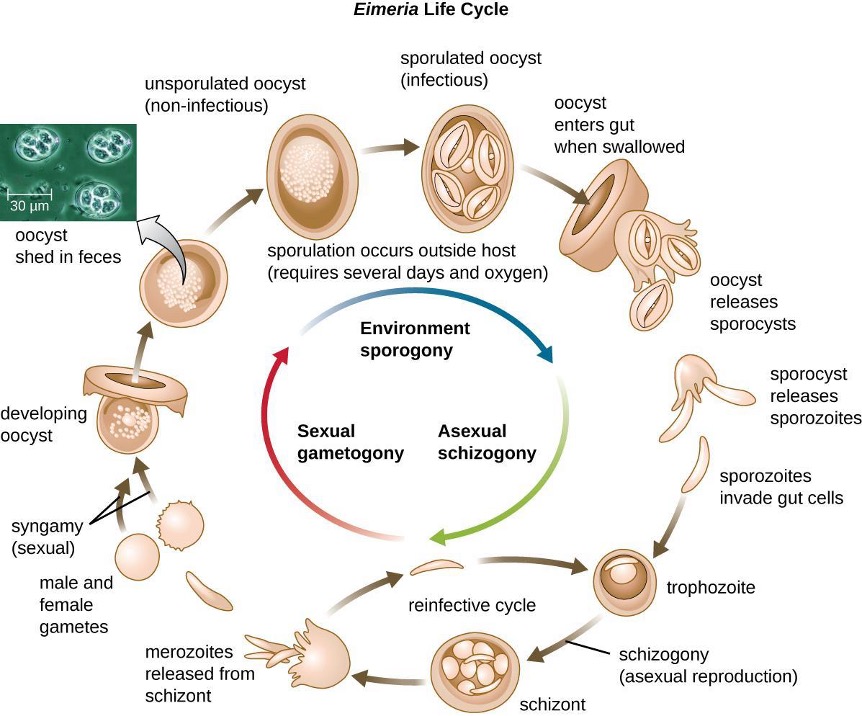
Protozoans have a variety of reproductive mechanisms. Some protozoans reproduce asexually and others reproduce sexually; still others are capable of both sexual and asexual reproduction. In protozoans, asexual reproduction occurs by binary fission, budding, or schizogony. In schizogony, the nucleus of a cell divides multiple times before the cell divides into many smaller cells. The products of schizogony are called merozoites and they are stored in structures known as schizonts. Protozoans may also reproduce sexually, which increases genetic diversity and can lead to complex life cycles. Protozoans can produce haploid gametes that fuse through syngamy. However, they can also exchange genetic material by joining to exchange DNA in a process called conjugation. This is a different process than the conjugation that occurs in bacteria. The term protist conjugation refers to a true form of eukaryotic sexual reproduction between two cells of different mating types. It is found in ciliates, a group of protozoans.
All protozoans have a plasma membrane, or plasmalemma, and some have bands of protein just inside the membrane that add rigidity, forming a structure called the pellicle. Some protists, including protozoans, have distinct layers of cytoplasm under the membrane. In these protists, the outer gel layer (with microfilaments of actin) is called the ectoplasm. Inside this layer is a sol (fluid) region of cytoplasm called the endoplasm. These structures contribute to complex cell shapes in some protozoans, whereas others (such as amoebas) have more flexible shapes (figure 2.46).
Different groups of protozoans have specialized feeding structures. They may have a specialized structure for taking in food through phagocytosis, called a cytostome, as well as a specialized structure for the exocytosis of wastes called a cytoproct. Oral grooves leading to cytostomes are lined with hair-like cilia to sweep in food particles. Protozoans are heterotrophic. Protozoans that are holozoic ingest whole food particles through phagocytosis. Forms that are saprozoic ingest small, soluble food molecules.
Many protists have whip-like flagella or hair-like cilia made of microtubules that can be used for locomotion (figure 2.46). Other protists use cytoplasmic extensions known as pseudopodia (“false feet”) to attach the cell to a surface; they then allow cytoplasm to flow into the extension, thus moving themselves forward.
Protozoans have a variety of unique organelles and sometimes lack organelles found in other cells. Some have contractile vacuoles, organelles that can be used to move water out of the cell for osmotic regulation (salt and water balance) (figure 2.46). Mitochondria may be absent in parasites or can be altered to kinetoplastids (modified mitochondria) or hydrogenosomes.
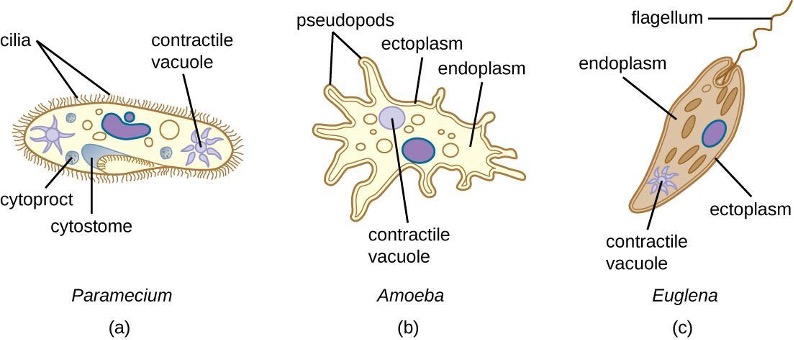
Table 2.16 lists the eukaryote supergroups and some examples.
Supergroup | Subgroups | Distinguishing Features | Examples | Clinical notes |
---|---|---|---|---|
Excavata | Fornicata | Form cysts Pair of equal nuclei No mitochondria Often parasitic Four free flagella |
Giardia lamblia | Giardiasis |
Parabasalids | No mitochondria Four free flagella One attached flagella No cysts Parasitic or symbiotic Basal bodies Kinetoplastids |
Trichomonas | Trichomoniasis | |
Euglenozoans | Photosynthetic or heterotrophic Flagella |
Euglena | N/a | |
Trypanosoma | African sleeping sickness, Chagas disease | |||
Leishmania | Leishmaniasis | |||
Chromalveolata | Dinoflagellates | Cellulose theca Two dissimilar flagella |
Gonyaulax | Red tides |
Alexandrium | Paralytic shellfish poisoning | |||
Pfiesteria | Harmful algal blooms | |||
Apicomplexans | Intracellular parasite Apical organelles |
Plasmodium | Malaria | |
Cryptosporidium | Cryptosporidiosis | |||
Theileria (Babesia) | Babesiosis | |||
Toxoplasma | Toxoplasmosis | |||
Ciliates | Cilia | Balantidium | Balantidiasis | |
Paramecium | N/a | |||
Stentor | N/a | |||
Oomycetes/peronosporomycetes | "Water molds" Generally diploid Cellulose cell walls |
Phytophthora | Disease in crops | |
Rhizaria | Formaninifera | Amoeboid Threadlike pseudopodia Calcium carbonate shells |
Astrolonche | N/a |
Radiolaria | Amoeboid Threadlike pseudopodia Silica shells |
Actinomma | N/a | |
Cercozoa | Amoeboid Threadlike pseudopodia Complex shells Parasitic forms |
Spongospora subterranea | Powdery scab (potato disease | |
Plasmodiophora brassicae | Cabbage clubroot | |||
Archaeplastida | Red algae | Chlorophyll a Phycoerythrin Phycocyanin Floridean starch Agar in cell walls |
Gelidium | Source of agar |
Gracilaria | Source of agar | |||
Chlorophytes | Chlorophyll a Chlorophyll b Cellulose cell walls Starch storage |
Acetabularia | N/a | |
Ulva | N/a | |||
Amoebozoa | Slime molds | Plasmodial and cellular forms | Dictyostelium | N/a |
Entamoebas | Trophozoites Form cysts |
Entamoeba | Amoebiasis | |
Naegleria | Primary amoebic meningoencephalitis | |||
Acanthamoeba | Keratitis, granulomatous amoebic encephalitis | |||
Opisthokonta | Fungi | Chitin cell walls Unicellular or multicellular Often hyphae |
Zygomycetes | Zygomycosis |
Ascomycetes | Candidiasis | |||
Basidiomycetes | Cryptococcosis | |||
Microsporidia | Microsporidiosis | |||
Animals | Multicellular heterotrophs No cell walls |
Nematoda | Trichinosis; hookworm and pinworm infections | |
Trematoda | Schistosomiasis | |||
Cestoda | Tapeworm infections |
Table 2.16: The eukaryote supergroups and some examples
Amoebozoa
The supergroup Amoebozoa includes protozoans that use amoeboid movement. Actin microfilaments produce pseudopodia, into which the remainder of the protoplasm flows, thereby moving the organism. The genus Entamoeba includes commensal or parasitic species, including the medically important E. histolytica, which is transmitted by cysts in feces and is the primary cause of amoebic dysentery. Another member of this group that is pathogenic to humans is Acanthamoeba, which can cause keratitis (corneal inflammation) and blindness. The notorious “brain eating amoeba,” Naegleria fowleri, is a considered a distant relative of the Amoebozoa and is classified in the phylum Percolozoa.
The Eumycetozoa are an unusual group of organisms called slime molds, which have previously been classified as animals, fungi, and plants (figure 2.47). Slime molds can be divided into two types: cellular slime molds and plasmodial slime molds. The cellular slime molds exist as individual amoeboid cells that periodically aggregate into a mobile slug. The aggregate then forms a fruiting body that produces haploid spores. Plasmodial slime molds exist as large, multinucleate amoeboid cells that form reproductive stalks to produce spores that divide into gametes. One cellular slime mold, Dictyostelium discoideum, has been an important study organism for understanding cell differentiation, because it has both single-celled and multicelled life stages, with the cells showing some degree of differentiation in the multicelled form. Figure 2.48 and figure 2.49 illustrate the life cycles of cellular and plasmodial slime molds, respectively.
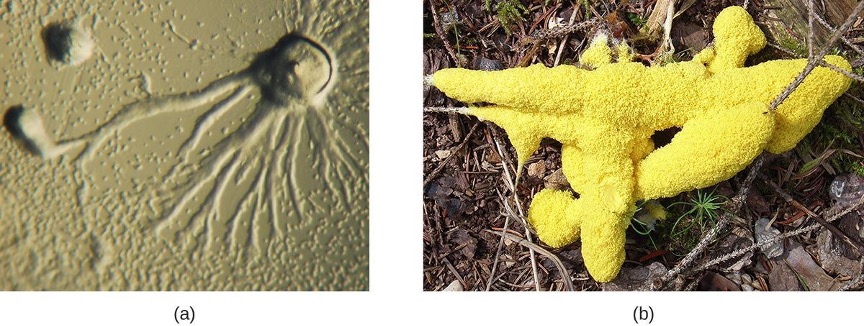
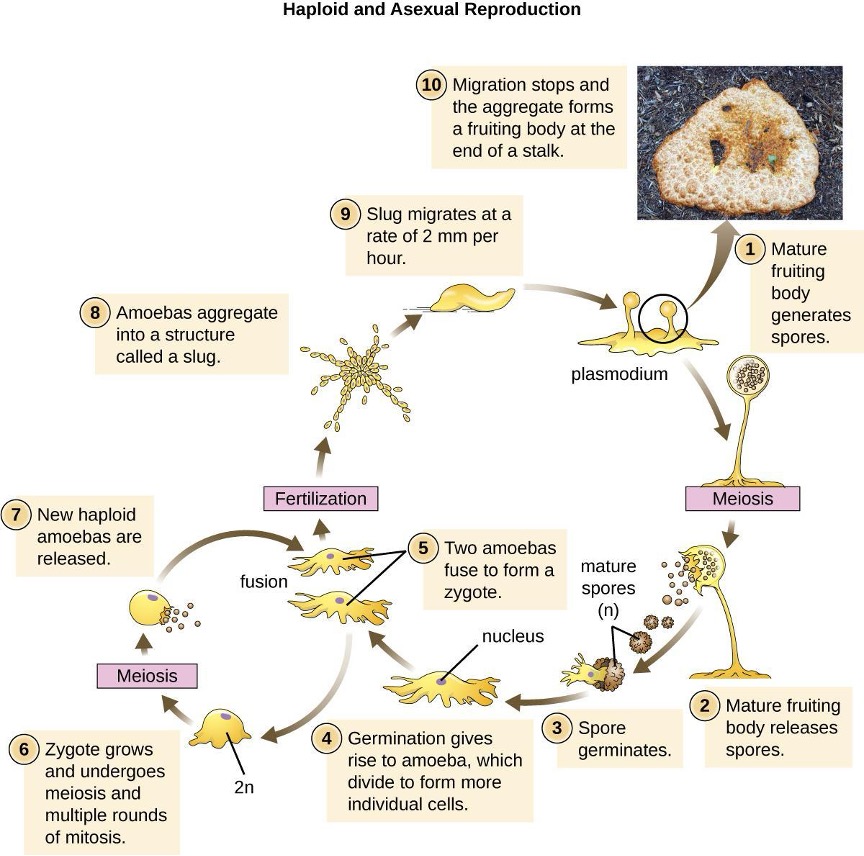
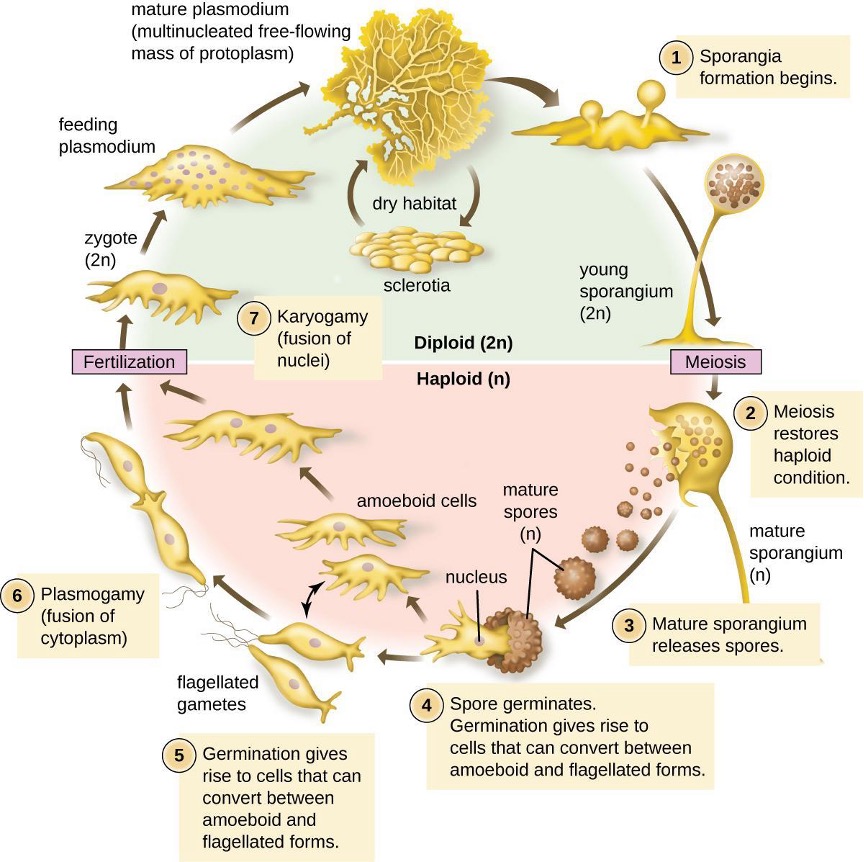
Chromalveolata
The supergroup Chromalveolata is united by similar origins of its members’ plastids and includes the apicomplexans, ciliates, diatoms, and dinoflagellates, among other groups. The apicomplexans are intra- or extracellular parasites that have an apical complex at one end of the cell. The apical complex is a concentration of organelles, vacuoles, and microtubules that allows the parasite to enter host cells (figure 2.50). Apicomplexans have complex life cycles that include an infective sporozoite that undergoes schizogony to make many merozoites (see the example in figure 2.45). Many are capable of infecting a variety of animal cells, from insects to livestock to humans, and their life cycles often depend on transmission between multiple hosts. The genus Plasmodium is an example of this group.
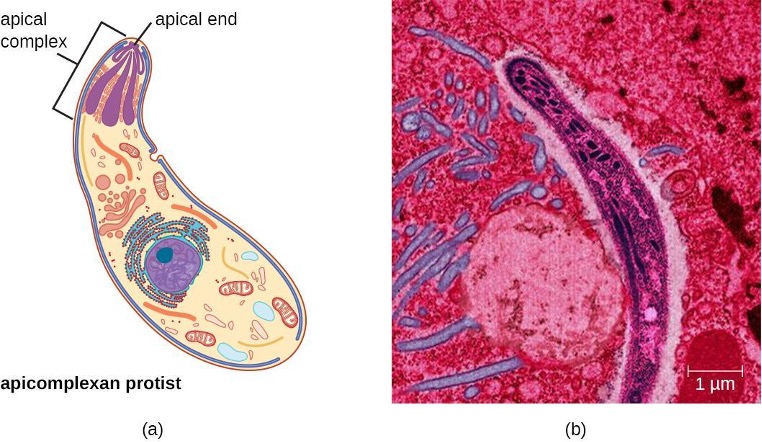
Other apicomplexans are also medically important. Cryptosporidium parvum causes intestinal symptoms and can cause epidemic diarrhea when the cysts contaminate drinking water. Theileria (Babesia) microti, transmitted by the tick Ixodes scapularis, causes recurring fever that can be fatal and is becoming a common transfusion-transmitted pathogen in the United States (Theileria and Babesia are closely related genera and there is some debate about the best classification). Finally, Toxoplasma gondii causes toxoplasmosis and can be transmitted from cat feces, unwashed fruit and vegetables, or from undercooked meat. Because toxoplasmosis can be associated with serious birth defects, pregnant women need to be aware of this risk and use caution if they are exposed to the feces of potentially infected cats. A national survey found the frequency of individuals with antibodies for toxoplasmosis (and thus who presumably have a current latent infection) in the United States to be 11%. Rates are much higher in other countries, including some developed countries.[17] There is also evidence and a good deal of theorizing that the parasite may be responsible for altering infected humans’ behavior and personality traits.[18]
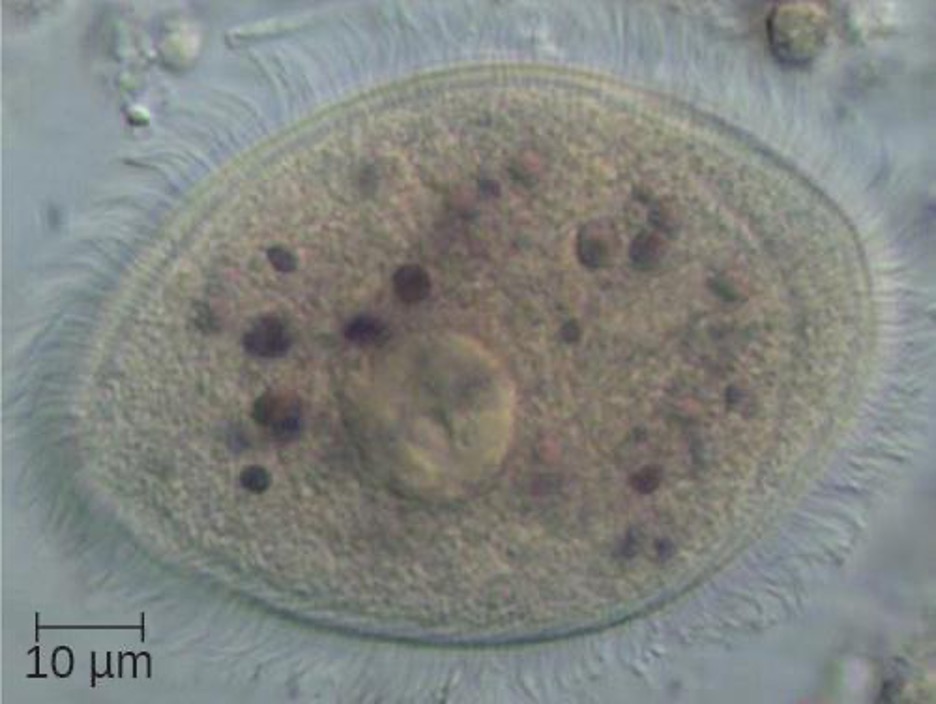
The ciliates (Ciliaphora), also within the Chromalveolata, are a large, very diverse group characterized by the presence of cilia on their cell surface. Although the cilia may be used for locomotion, they are often used for feeding as well, and some forms are nonmotile. Balantidium coli (figure 2.51) is the only parasitic ciliate that affects humans by causing intestinal illness, although it rarely causes serious medical issues except in the immunocompromised (those having a weakened immune system). Perhaps the most familiar ciliate is Paramecium, a motile organism with a clearly visible cytostome and cytoproct that is often studied in biology laboratories (figure 2.52). Another ciliate, Stentor, is sessile and uses its cilia for feeding (figure 2.53). Generally, these organisms have a micronucleus that is diploid, somatic, and used for sexual reproduction by conjugation. They also have a macronucleus that is derived from the micronucleus; the macronucleus becomes polyploid (multiple sets of duplicate chromosomes), and has a reduced set of metabolic genes.
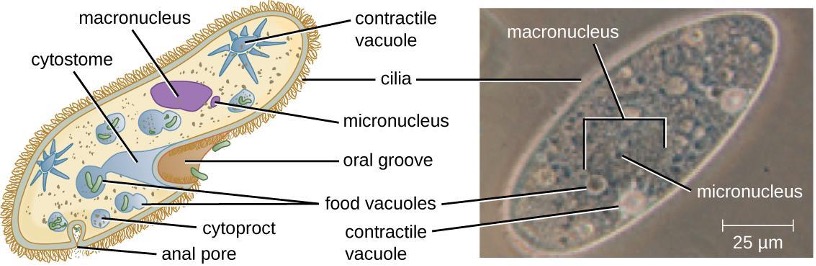
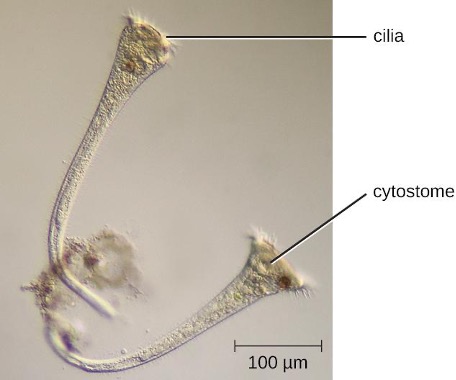
Ciliates are able to reproduce through conjugation, in which two cells attach to each other. In each cell, the diploid micronuclei undergo meiosis, producing eight haploid nuclei each. Then, all but one of the haploid micronuclei and the macronucleus disintegrate; the remaining (haploid) micronucleus undergoes mitosis. The two cells then exchange one micronucleus each, which fuses with the remaining micronucleus to form a new, genetically different, diploid micronucleus. The diploid micronucleus undergoes two mitotic divisions, so each cell has four micronuclei. Two of these four micronuclei then combine to form a new macronucleus. The chromosomes in the macronucleus then replicate repeatedly, the macronucleus reaches its polyploid state, and the two cells separate. The two cells are now genetically different from each other and from their previous versions.
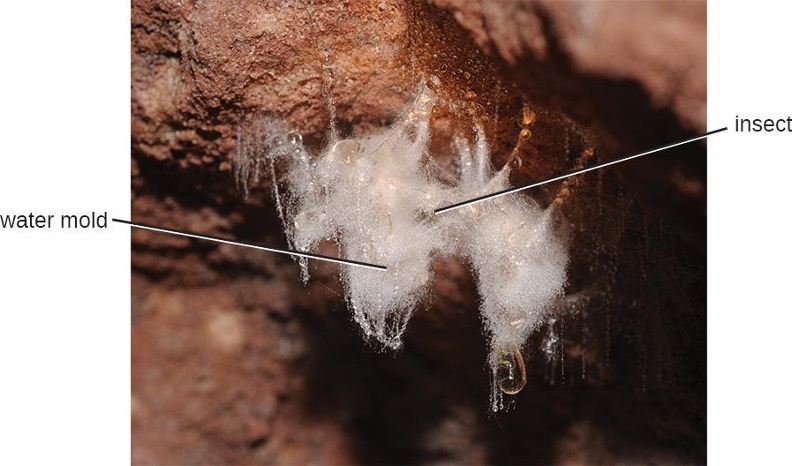
Öomycetes, also called water molds, have similarities to fungi and were once classified with them. However, they differ from fungi in several important ways. Unlike the chitinous cell walls of fungi, Öomycetes have walls composed of cellulose. Additionally, they are generally diploid, whereas the dominant life forms of fungi are typically haploid. Phytophthora, the plant pathogen found in the soil that caused the Irish potato famine, is classified within this group (figure 2.54).
Excavata
The third and final supergroup to be considered in this section is the Excavata, which includes primitive eukaryotes and many parasites with limited metabolic abilities. These organisms have complex cell shapes and structures, often including a depression on the surface of the cell called an excavate. The group Excavata includes the subgroups Fornicata, Parabasalia, and Euglenozoa. The Fornicata lack mitochondria but have flagella. This group includes Giardia lamblia (also known as G. intestinalis or G. duodenalis), a widespread pathogen that causes diarrheal illness and can be spread through cysts from feces that contaminate water supplies (figure 2.44). Parabasalia are frequent animal endosymbionts; they live in the guts of animals like termites and cockroaches. They have basal bodies and modified mitochondria (kinetoplastids). They also have a large, complex cell structure with an undulating membrane and often have many flagella. The trichomonads (a subgroup of the Parabasalia) include pathogens such as Trichomonas vaginalis, which causes the human sexually transmitted disease trichomoniasis. Trichomoniasis often does not cause symptoms in men, but men are able to transmit the infection. In women, it causes vaginal discomfort and discharge and may cause complications in pregnancy if left untreated.
The Euglenozoa are common in the environment and include photosynthetic and nonphotosynthetic species. Members of the genus Euglena are typically not pathogenic. Their cells have two flagella, a pellicle, a stigma (eyespot) to sense light, and chloroplasts for photosynthesis (figure 2.55). The pellicle of Euglena is made of a series of protein bands surrounding the cell; it supports the cell membrane and gives the cell shape.
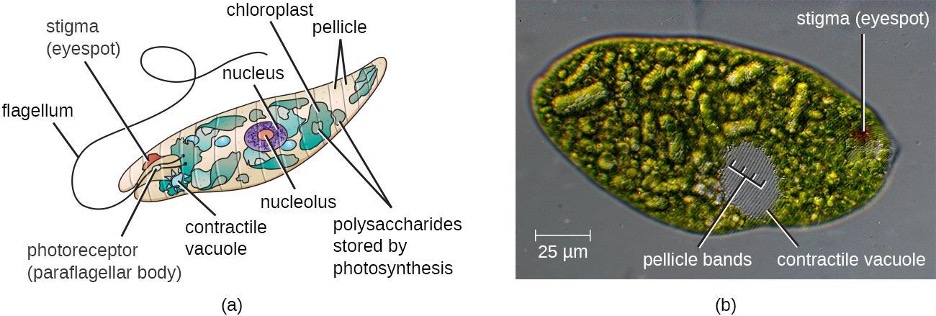
The Euglenozoa also include the trypanosomes, which are parasitic pathogens. The genus Trypanosoma includes T. brucei, which causes African trypanosomiasis (African sleeping sickness and T. cruzi, which causes American trypanosomiasis (Chagas disease). These tropical diseases are spread by insect bites. In African sleeping sickness, T. brucei colonizes the blood and the brain after being transmitted via the bite of a tsetse fly (Glossina spp.) (figure 2.56). The early symptoms include confusion, difficulty sleeping, and lack of coordination. Left untreated, it is fatal.
Chagas’ disease originated and is most common in Latin America. The disease is transmitted by Triatoma spp., insects often called “kissing bugs,” and affects either the heart tissue or tissues of the digestive system. Untreated cases can eventually lead to heart failure or significant digestive or neurological disorders.
The genus Leishmania includes trypanosomes that cause disfiguring skin disease and sometimes systemic illness as well.
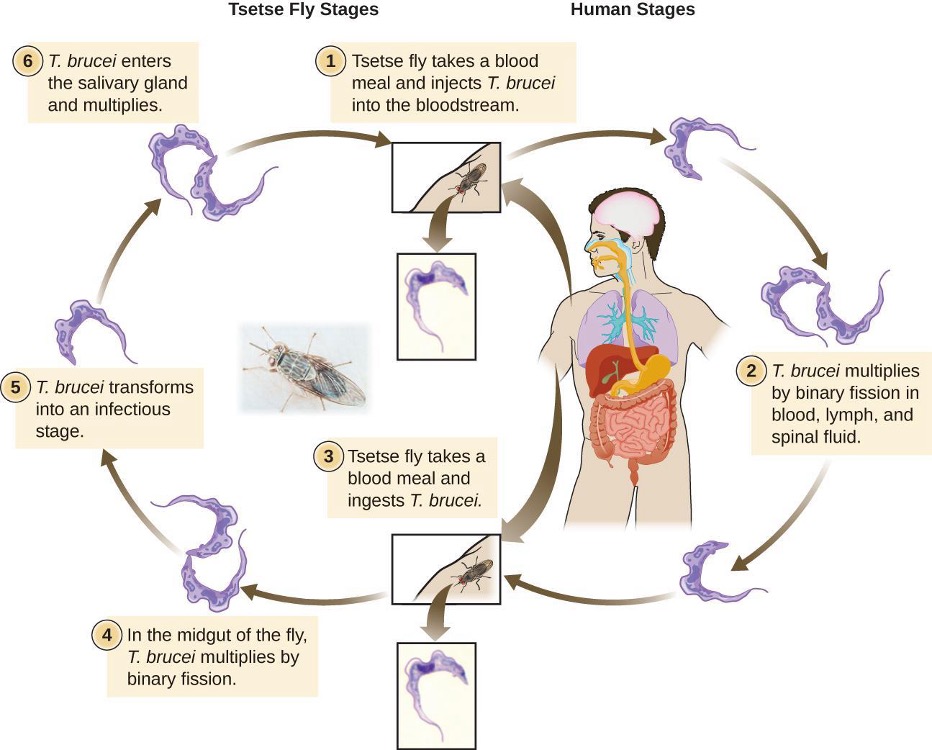
2.8 Parasitic Helminths
There are two major groups of parasitic helminths: the roundworms (Nematoda) and flatworms (Platyhelminthes). Of the many species that exist in these groups, about half are parasitic and some are important human pathogens. As animals, they are multicellular and have organ systems. However, the parasitic species often have limited digestive tracts, nervous systems, and locomotor abilities. Parasitic forms may have complex reproductive cycles with several different life stages and more than one type of host. Some are monoecious, having both male and female reproductive organs in a single individual, while others are dioecious, each having either male or female reproductive organs.
Nematoda (Roundworms)
Phylum Nematoda (the roundworms) is a diverse group containing more than 15,000 species, of which several are notable human parasites (figure 2.57). These unsegmented worms have a full digestive system even when parasitic. Some are common intestinal parasites, and their eggs can sometimes be identified in feces or around the anus of infected individuals. Ascaris lumbricoides is the largest nematode intestinal parasite found in humans; females may reach lengths greater than 1 meter. A. lumbricoides is also very widespread, even in developed nations, although it is now a relatively uncommon problem in the United States. It may cause symptoms ranging from relatively mild (such as a cough and mild abdominal pain) to severe (such as intestinal blockage and impaired growth).
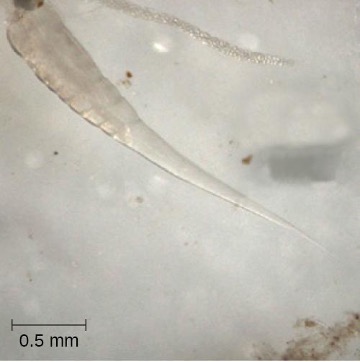
Of all nematode infections in the United States, pinworm (caused by Enterobius vermicularis) is the most common. Pinworm causes sleeplessness and itching around the anus, where the female worms lay their eggs during the night. Toxocara canis and T. cati are nematodes found in dogs and cats, respectively, that can be transmitted to humans, causing toxocariasis. Antibodies to these parasites have been found in approximately 13.9% of the U.S. population, suggesting that exposure is common.[19] Infection can cause larval migrans, which can result in vision loss and eye inflammation, or fever, fatigue, coughing, and abdominal pain, depending on whether the organism infects the eye or the viscera. Another common nematode infection is hookworm, which is caused by Necator americanus (the New World or North American hookworm) and Ancylostoma duodenale (the Old World hookworm). Symptoms of hookworm infection can include abdominal pain, diarrhea, loss of appetite, weight loss, fatigue, and anemia.
Trichinellosis, also called trichinosis, caused by Trichinella spiralis, is contracted by consuming undercooked meat, which releases the larvae and allows them to encyst in muscles. Infection can cause fever, muscle pains, and digestive system problems; severe infections can lead to lack of coordination, breathing and heart problems, and even death. Finally, heartworm in dogs and other animals is caused by the nematode Dirofilaria immitis, which is transmitted by mosquitoes. Symptoms include fatigue and cough; when left untreated, death may result.
Platyhelminthes (Flatworms)
Phylum Platyhelminthes (the platyhelminthes) are flatworms. This group includes the flukes, tapeworms, and the turbellarians, which include planarians. The flukes and tapeworms are medically relevant parasites (figure 2.58).
The flukes (trematodes) are nonsegmented flatworms that have an oral sucker (figure 2.59) (and sometimes a second ventral sucker) and attach to the inner walls of intestines, lungs, large blood vessels, or the liver. Trematodes have complex life cycles, often with multiple hosts. Several important examples are the liver flukes (Clonorchis and Opisthorchis), the intestinal fluke (Fasciolopsis buski), and the oriental lung fluke (Paragonimus westermani). Schistosomiasis is a serious parasitic disease, considered second in the scale of its impact on human populations only to malaria. The parasites Schistosoma mansoni, S. haematobium, and S. japonicum (found in freshwater snails) are responsible for schistosomiasis. Immature forms burrow through the skin into the blood. They migrate to the lungs, then to the liver and, later, other organs. Symptoms include anemia, malnutrition, fever, abdominal pain, fluid buildup, and sometimes death. Figure 2.60 presents the life cycle of Schistosoma spp.
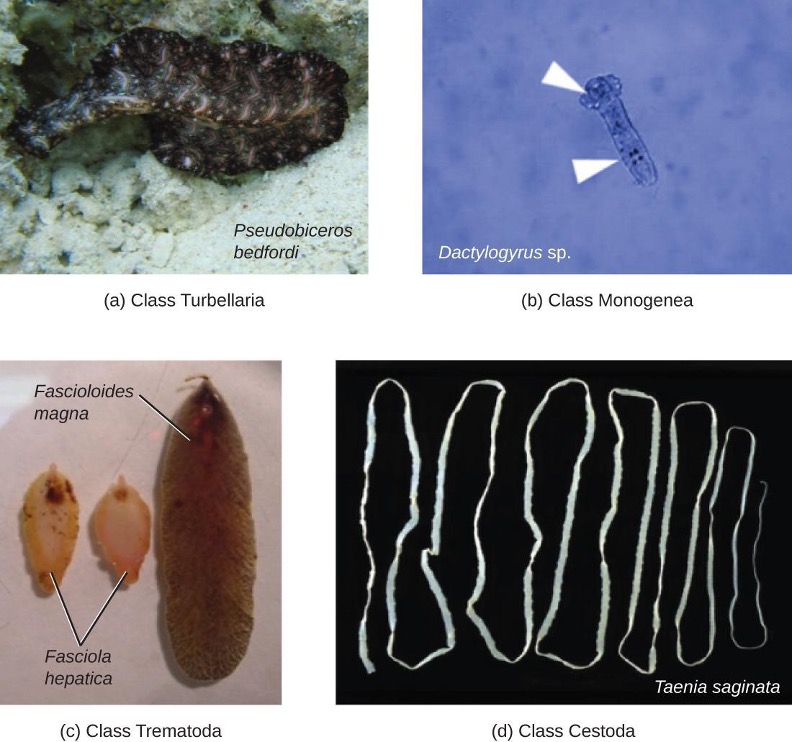
The other medically important group of platyhelminths are commonly known as tapeworms (cestodes) and are segmented flatworms that may have suckers or hooks at the scolex (head region) (figure 2.59). Tapeworms use these suckers or hooks to attach to the wall of the small intestine. The body of the worm is made up of segments called proglottids that contain reproductive structures; these detach when the gametes are fertilized, releasing gravid proglottids with eggs. Tapeworms often have an intermediate host that consumes the eggs, which then hatch into a larval form called an oncosphere. The oncosphere migrates to a particular tissue or organ in the intermediate host, where it forms cysticerci. After being eaten by the definitive host, the cysticerci develop into adult tapeworms in the host’s digestive system (figure 2.61). Taenia saginata (the beef tapeworm) and T. solium (the pork tapeworm) enter humans through ingestion of undercooked, contaminated meat. The adult worms develop and reside in the intestine, but the larval stage may migrate and be found in other body locations such as skeletal and smooth muscle. The beef tapeworm is relatively benign, although it can cause digestive problems and, occasionally, allergic reactions. The pork tapeworm can cause more serious problems when the larvae leave the intestine and colonize other tissues, including those of the central nervous system. Diphylobothrium latum is the largest human tapeworm and can be ingested in undercooked fish. It can grow to a length of 15 meters. Echinococcus granulosus, the dog tapeworm, can parasitize humans and uses dogs as a host.
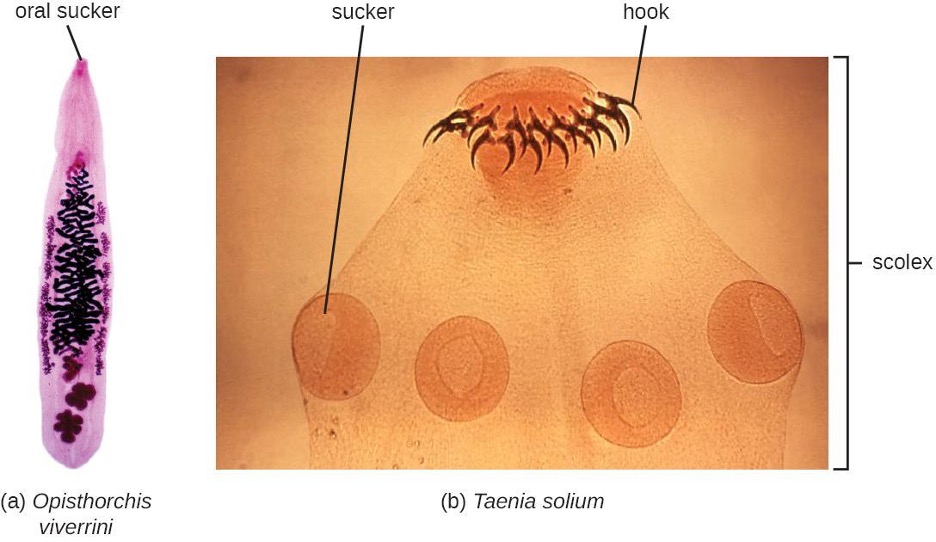
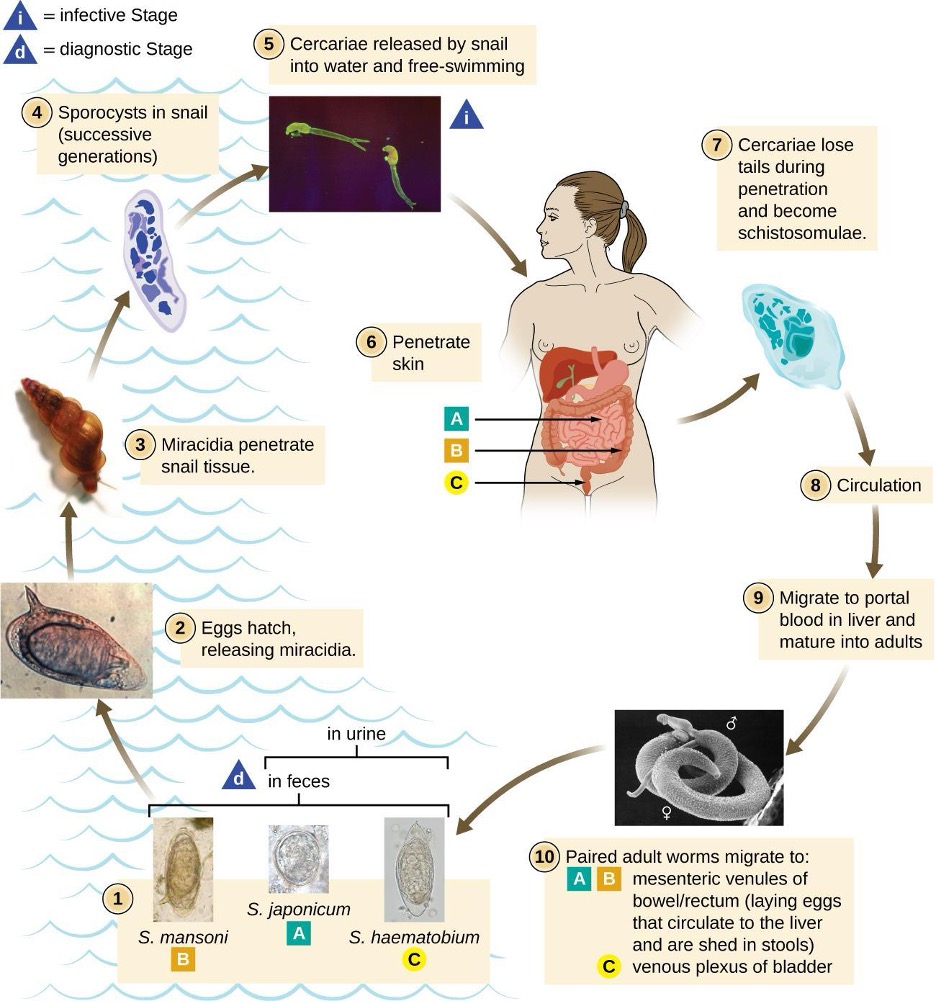
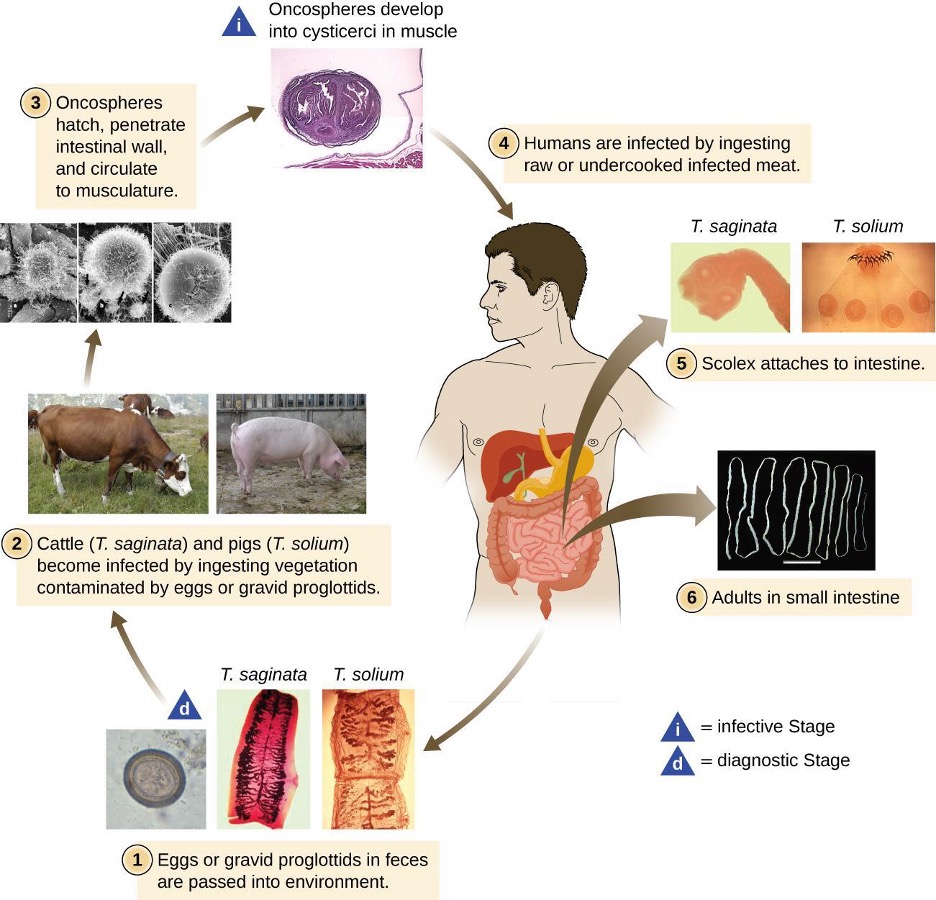
2.9 Fungi
Fungi are important to humans in a variety of ways. Both microscopic and macroscopic fungi have medical relevance, with some pathogenic species that can cause mycoses (illnesses caused by fungi). Some pathogenic fungi are opportunistic, meaning that they mainly cause infections when the host’s immune defenses are compromised and do not normally cause illness in healthy individuals. Fungi are important in other ways as well. They act as decomposers in the environment, and they are critical for the production of certain foods such as cheeses. Fungi are also major sources of antibiotics, such as penicillin from the fungus Penicillium.
Characteristics of Fungi
Fungi have well-defined characteristics that set them apart from other organisms. Most multicellular fungal bodies, commonly called molds, are made up of filaments called hyphae. Hyphae can form a tangled network called a mycelium and form the thallus (body) of fleshy fungi. Hyphae that have walls between the cells are called septate hyphae; hyphae that lack walls and cell membranes between the cells are called nonseptate or coenocytic hyphae) (figure 2.62).
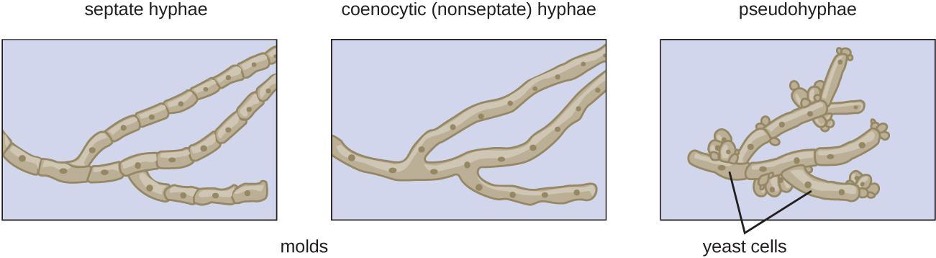
In contrast to molds, yeasts are unicellular fungi. The budding yeasts reproduce asexually by budding off a smaller daughter cell; the resulting cells may sometimes stick together as a short chain or pseudohyphae (figure 2.62).
Some fungi are dimorphic, meaning they have more than one appearance during their life cycle. These dimorphic fungi may be able to appear as yeasts or molds, which can be important for infectivity. They are capable of changing their appearance in response to environmental changes such as nutrient availability or fluctuations in temperature, growing as a mold, for example, at 25 °C (77 °F), and as yeast cells at 37 °C (98.6 °F). This ability helps dimorphic fungi to survive in diverse environments. Two examples of dimorphic yeasts are the human pathogens Histoplasma capsulatum and Candida albicans. H. capsulatum causes the lung disease histoplasmosis, and C. albicans is associated with vaginal yeast infections, oral thrush, and candidiasis of the skin (figure 2.63).
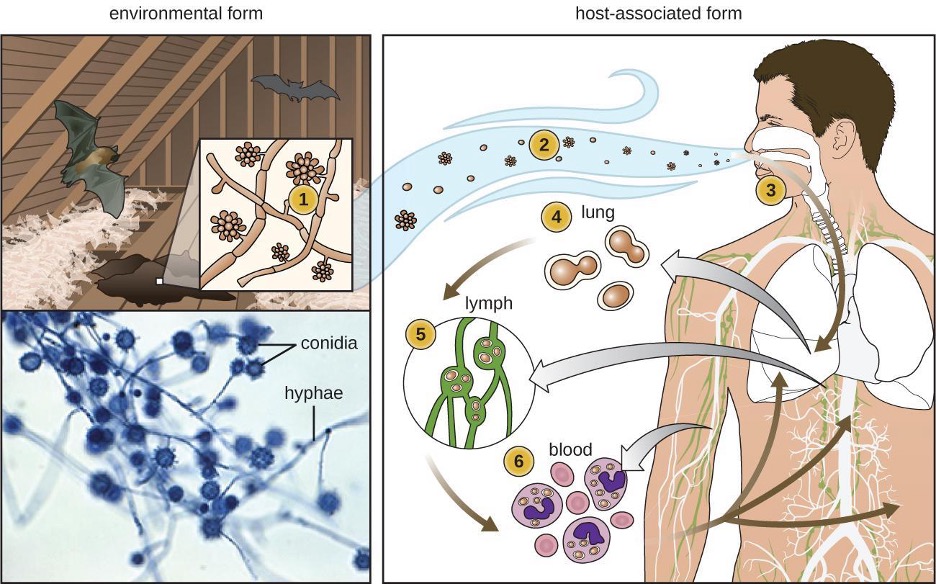
There are notable unique features in fungal cell walls and membranes. Fungal cell walls contain chitin, as opposed to the cellulose found in the cell walls of plants and many protists. Additionally, whereas animals have cholesterol in their cell membranes, fungal cell membranes have different sterols called ergosterols. Ergosterols are often exploited as targets for antifungal drugs.
Fungal life cycles are unique and complex. Fungi reproduce sexually either through cross- or self-fertilization. Haploid fungi form hyphae that have gametes at the tips. Two different mating types (represented as + type and – type) are involved. The cytoplasms of the + and – type gametes fuse (in an event called plasmogamy), producing a cell with two distinct nuclei (a dikaryotic cell). Later, the nuclei fuse (in an event called karyogamy) to create a diploid zygote. The zygote undergoes meiosis to form spores that germinate to start the haploid stage, which eventually creates more haploid mycelia (figure 2.64). Depending on the taxonomic group, these sexually produced spores are known as zygospores (in Zygomycota), ascospores (in Ascomycota), or basidiospores (in Basidiomycota) (figure 2.65).
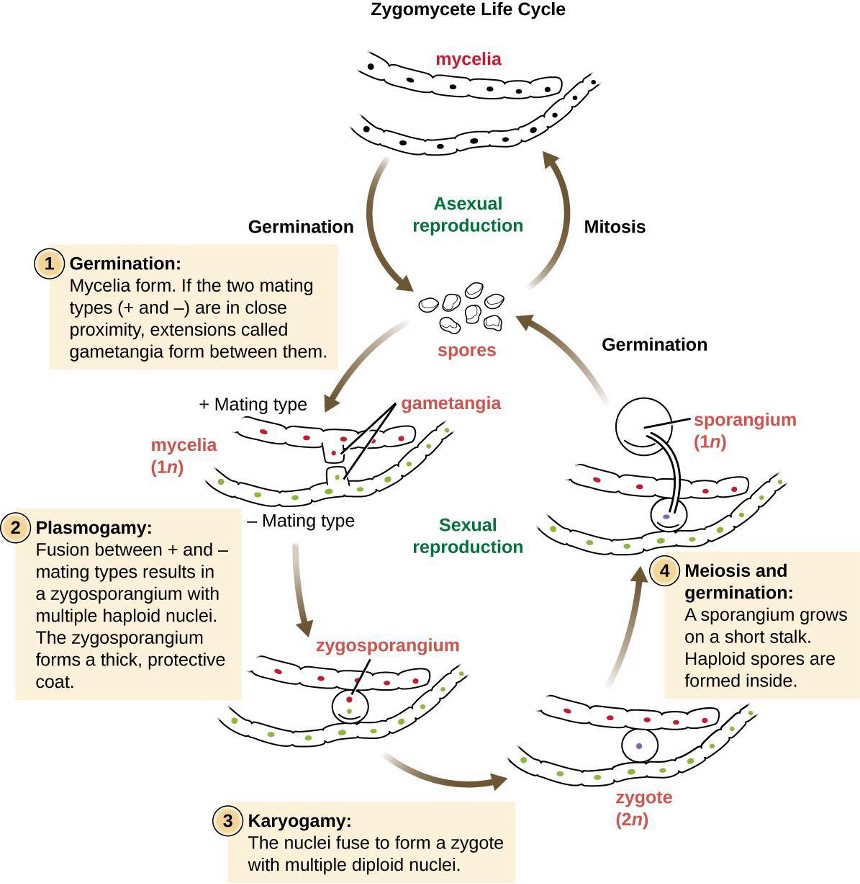
Fungi may also exhibit asexual reproduction by mitosis, mitosis with budding, fragmentation of hyphae, and formation of asexual spores by mitosis. These spores are specialized cells that, depending on the organism, may have unique characteristics for survival, reproduction, and dispersal. Fungi exhibit several types of asexual spores and these can be important in classification.
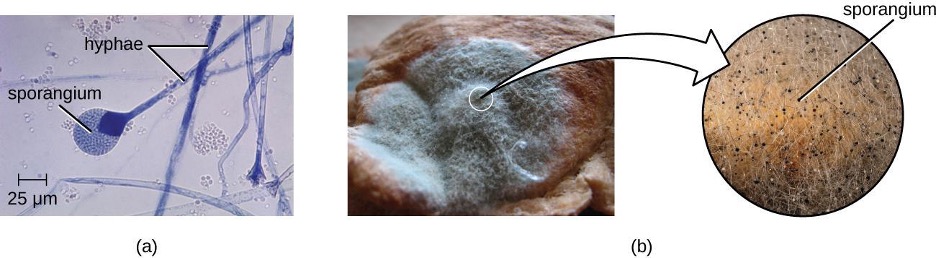
Fungal Diversity
The fungi are very diverse, comprising seven major groups. Not all of the seven groups contain pathogens. Some of these groups are generally associated with plants and include plant pathogens. For example, Urediniomycetes and Ustilagomycetes include the plant rusts and smuts, respectively. These form reddish or dark masses, respectively, on plants as rusts (red) or smuts (dark). Some species have substantial economic impact because of their ability to reduce crop yields. Glomeromycota includes the mycorrhizal fungi, important symbionts with plant roots that can promote plant growth by acting like an extended root system. The Glomeromycota are obligate symbionts, meaning that they can only survive when associated with plant roots; the fungi receive carbohydrates from the plant and the plant benefits from the increased ability to take up nutrients and minerals from the soil. The Chytridiomycetes (chytrids) are small fungi, but are extremely ecologically important. Chytrids are generally aquatic and have flagellated, motile gametes; specific types are implicated in amphibian declines around the world. Because of their medical importance, we will focus on Zygomycota, Ascomycota, Basidiomycota, and Microsporidia. Table 2.17 summarizes the characteristics of these medically important groups of fungi.
The Zygomycota (zygomycetes) are mainly saprophytes with coenocytic hyphae and haploid nuclei. They use sporangiospores for asexual reproduction. The group name comes from the zygospores that they use for sexual reproduction (figure 2.64), which have hard walls formed from the fusion of reproductive cells from two individuals. Zygomycetes are important for food science and as crop pathogens. One example is Rhizopus stolonifer (figure 2.65), an important bread mold that also causes rice seedling blight. Mucor is a genus of fungi that can potentially cause necrotizing infections in humans, although most species are intolerant of temperatures found in mammalian bodies (figure 2.65).
The Ascomycota include fungi that are used as food (edible mushrooms, morels, and truffles), others that are common causes of food spoilage (bread molds and plant pathogens), and still others that are human pathogens. Ascomycota may have septate hyphae and cup-shaped fruiting bodies called ascocarps. Some genera of Ascomycota use sexually produced ascospores as well as asexual spores called conidia, but sexual phases have not been discovered or described for others. Some produce an ascus containing ascospores within an ascocarp (figure 2.66).
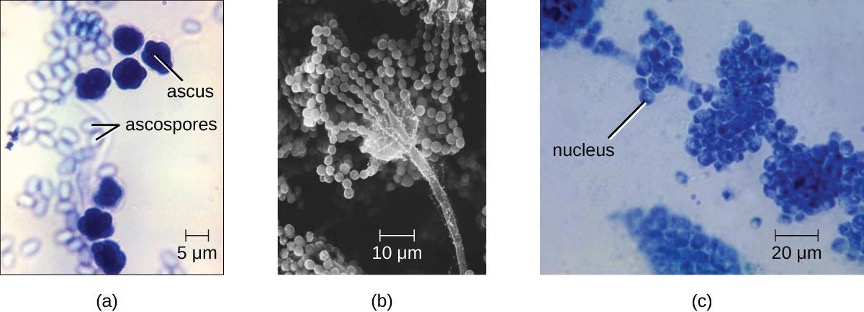
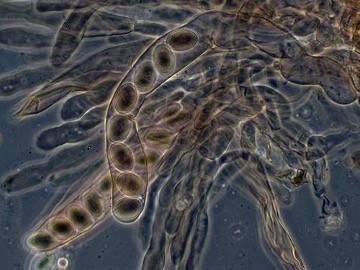
Examples of the Ascomycota include several bread molds and minor pathogens, as well as species capable of causing more serious mycoses. Species in the genus Aspergillus are important causes of allergy and infection, and are useful in research and in the production of certain fermented alcoholic beverages such as Japanese sake. The fungus Aspergillus flavus, a contaminant of nuts and stored grains, produces an aflatoxin that is both a toxin and the most potent known natural carcinogen. Neurospora crassa is of particular use in genetics research because the spores produced by meiosis are kept inside the ascus in a row that reflects the cell divisions that produced them, giving a direct view of segregation and assortment of genes (figure 2.67). Penicillium produces the antibiotic penicillin (figure 2.66).
Many species of ascomycetes are medically important. A large number of species in the genera Trichophyton, Microsporum, and Epidermophyton are dermatophytes, pathogenic fungi capable of causing skin infections such as athlete’s foot, jock itch, and ringworm (figure 2.42). Blastomyces dermatitidis is a dimorphic fungus that can cause blastomycosis, a respiratory infection that, if left untreated, can become disseminated to other body sites, sometimes leading to death. Another important respiratory pathogen is the dimorphic fungus Histoplasma capsulatum (figure 2.63), which is associated with birds and bats in the Ohio and Mississippi river valleys. Coccidioides immitis causes the serious lung disease Valley fever. Candida albicans, the most common cause of vaginal and other yeast infections, is also an ascomycete fungus; it is a part of the normal microbiota of the skin, intestine, genital tract, and ear (figure 2.66). Ascomycetes also cause plant diseases, including ergot infections, Dutch elm disease, and powdery mildews.
Saccharomyces yeasts, including the baker’s yeast S. cerevisiae, are unicellular ascomycetes with haploid and diploid stages (figure 2.68). This and other Saccharomyces species are used for brewing beer.
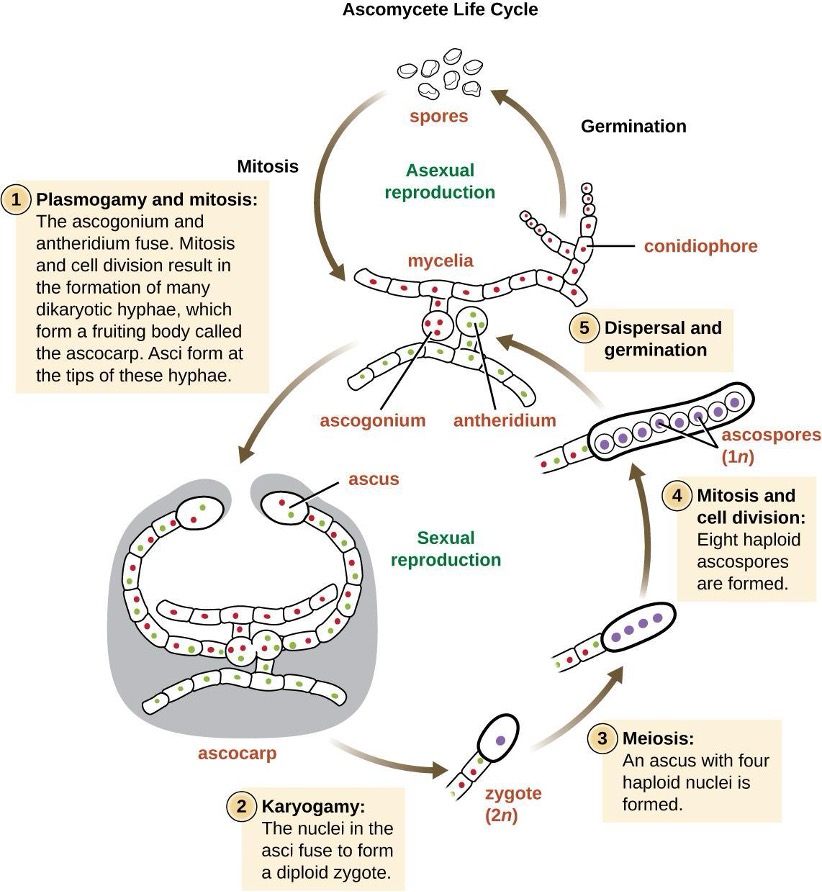
The Basidiomycota (basidiomycetes) are fungi that have basidia (club-shaped structures) that produce basidiospores (spores produced through budding) within fruiting bodies called basidiocarps (figure 2.69). They are important as decomposers and as food. This group includes rusts, stinkhorns, puffballs, and mushrooms. Several species are of particular importance. Cryptococcus neoformans, a fungus commonly found as a yeast in the environment, can cause serious lung infections when inhaled by individuals with weakened immune systems. The edible meadow mushroom, Agricus campestris, is a basidiomycete, as is the poisonous mushroom Amanita phalloides, known as the death cap. The deadly toxins produced by A. phalloides have been used to study transcription.
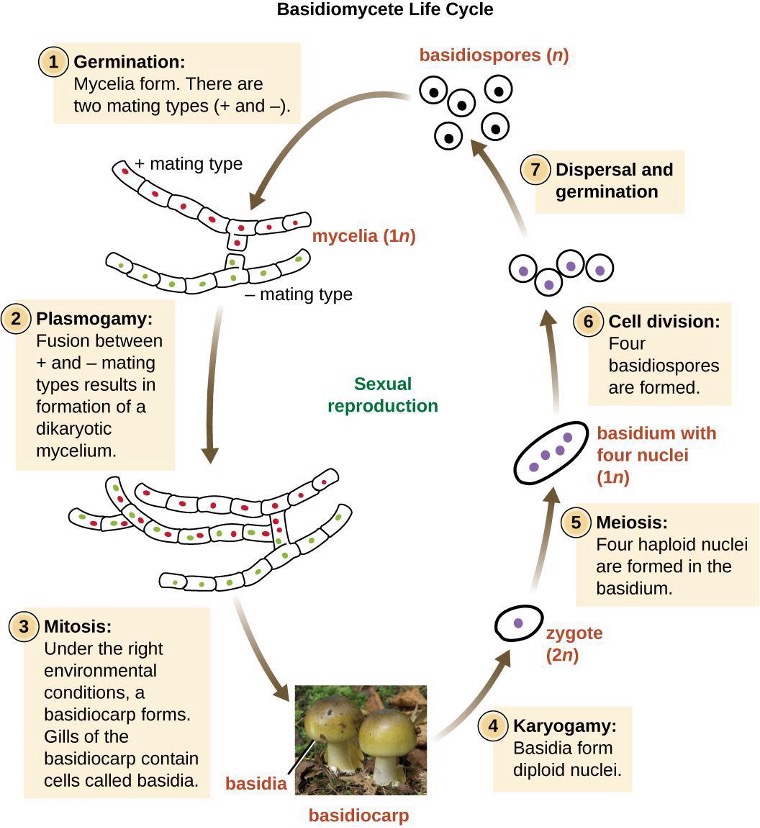
Finally, the Microsporidia are unicellular fungi that are obligate intracellular parasites. They lack mitochondria, peroxisomes, and centrioles, but their spores release a unique polar tubule that pierces the host cell membrane to allow the fungus to gain entry into the cell. A number of microsporidia are human pathogens, and infections with microsporidia are called microsporidiosis. One pathogenic species is Enterocystozoan bieneusi, which can cause symptoms such as diarrhea, cholecystitis (inflammation of the gall bladder), and in rare cases, respiratory illness.
Group | Characteristics | Examples | Medically Important Species | Image |
---|---|---|---|---|
Ascomycota | Septate hyphae Ascus with ascospores in ascocarp Condidiospores |
Cup fungi Edible mushrooms Morels Truffles Neurospora Penicillium |
Aspergillus spp. Trichophyton spp. Microsporum spp. Epidermonphyton spp. Blastomyces dermititus Histoplasma capsulatum |
![]() Aspergillus niger |
Basidiomycota | Basidia produce basidiospores in a basidiocarp | Club fungi Rusts Stinkhorns Puffballs Mushrooms Cryptococcus neoformans Amanita phalloides |
Crytococcus neoformans | ![]() Amanita phalloides |
Microsporidia | Lack mitochondria, peroxisomes, centrioles Spores produce a polar tube |
Enterocystozoan bieneusi | Enterocystozoan bieneusi | ![]() Microsporidia (unidentified) |
Zygomycota | Mainly saprophytes Coenocytic hyphae Haploid nuclei Zygospores |
Rhizopus stolonifera | Mucor spp. | ![]() Rhizopus sp. |
Table 2.17: Select groups of fungi
2.10 Viruses
Viruses are distinct biological entities; however, their evolutionary origin is still a matter of speculation. In terms of taxonomy, they are not included in the tree of life because they are acellular (not consisting of cells). In order to survive and reproduce, viruses must infect a cellular host, making them obligate intracellular parasites. The genome of a virus enters a host cell and directs the production of the viral components, proteins and nucleic acids, needed to form new virus particles called virions. New virions are made in the host cell by assembly of viral components. The new virions transport the viral genome to another host cell to carry out another round of infection. Table 2.18 summarizes the properties of viruses.
Characteristics of Viruses |
---|
Infectious, acellular pathogens |
Obligate intracellular parasites with host and cell-type specificity |
DNA or RNA genome (never both) |
Genome is surrounded by a protein capsid and, in some cases, a phospholipid membrane studded with viral glycoproteins |
Lack genes for many products needed for successful reproduction, requiring exploitation of host-cell genomes to reproduce |
Table 2.18: Characteristics of viruses
Hosts and Viral Transmission
Viruses can be transmitted through direct contact, indirect contact with fomites, or through a vector: an animal that transmits a pathogen from one host to another. Arthropods such as mosquitoes, ticks, and flies, are typical vectors for viral diseases, and they may act as mechanical vectors or biological vectors. Mechanical transmission occurs when the arthropod carries a viral pathogen on the outside of its body and transmits it to a new host by physical contact. Biological transmission occurs when the arthropod carries the viral pathogen inside its body and transmits it to the new host through biting.
Viruses that can be transmitted from an animal host to a human host can cause zoonoses. For example, the avian influenza virus originates in birds but can cause disease in humans. Reverse zoonoses are caused by infection of an animal by a virus that originated in a human.
Viral Structures
In general, virions (viral particles) are small and cannot be observed using a regular light microscope. They are much smaller than prokaryotic and eukaryotic cells; this is an adaptation allowing viruses to infect these larger cells (see figure 2.70). The size of a virion can range from 20 nm for small viruses up to 900 nm for typical, large viruses (see figure 2.72). Recent discoveries, however, have identified new giant viral species, such as Pandoravirus salinus and Pithovirus sibericum, with sizes approaching that of a bacterial cell.[20]
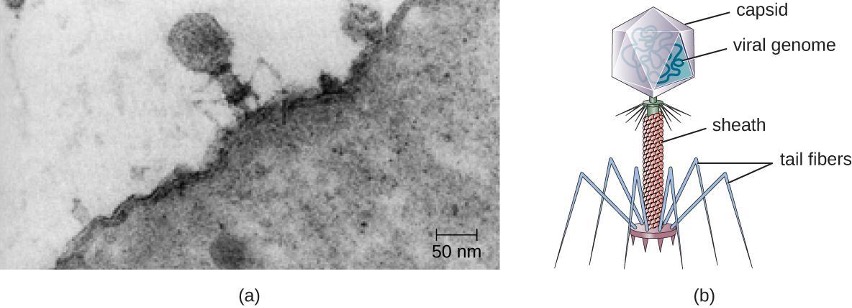
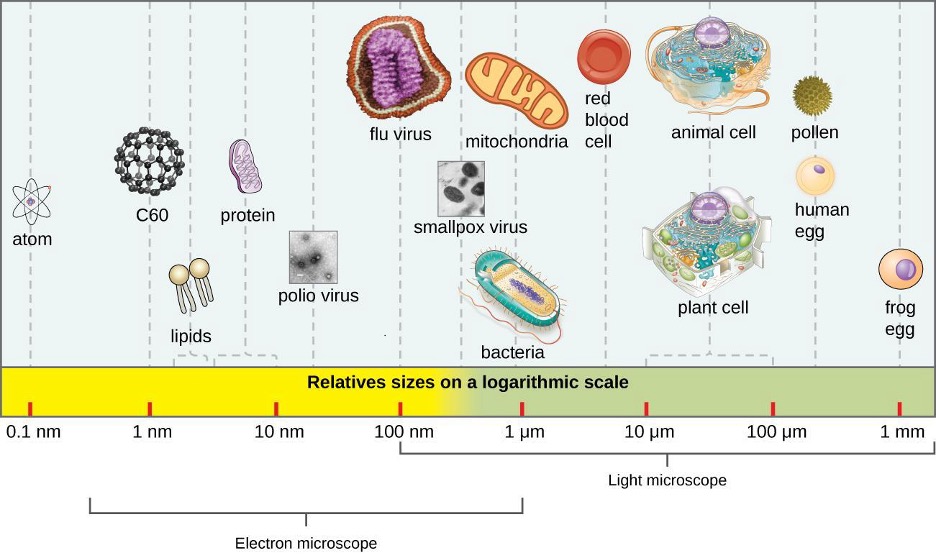
There are two categories of viruses based on general composition. Viruses formed from only a nucleic acid and capsid are called naked viruses or nonenveloped viruses. Viruses formed with a nucleic-acid packed capsid surrounded by a lipid layer are called enveloped viruses (see figure 2.72). The viral envelope is a small portion of phospholipid membrane obtained as the virion buds from a host cell. The viral envelope may either be intracellular or cytoplasmic in origin.
Extending outward and away from the capsid on some naked viruses and enveloped viruses are protein structures called spikes. At the tips of these spikes are structures that allow the virus to attach and enter a cell, like the influenza virus hemagglutinin spikes (H) or enzymes like the neuraminidase (N) influenza virus spikes that allow the virus to detach from the cell surface during release of new virions. Influenza viruses are often identified by their H and N spikes. For example, H1N1 influenza viruses were responsible for the pandemics in 1918 and 2009,[21] H2N2 for the pandemic in 1957, and H3N2 for the pandemic in 1968.
Viruses vary in the shape of their capsids, which can be either helical, polyhedral, or complex. A helical capsid forms the shape of tobacco mosaic virus (TMV), a naked helical virus, and Ebola virus, an enveloped helical virus. The capsid is cylindrical or rod shaped, with the genome fitting just inside the length of the capsid. Polyhedral capsids form the shapes of poliovirus and rhinovirus, and they consist of a nucleic acid surrounded by a polyhedral (many-sided) capsid in the form of an icosahedron. An icosahedral capsid is a three-dimensional, 20-sided structure with 12 vertices. These capsids somewhat resemble a soccer ball. Both helical and polyhedral viruses can have envelopes. Viral shapes seen in certain types of bacteriophages, such as T4 phage, and poxviruses like vaccinia virus, may have features of both polyhedral and helical viruses so they are described as a complex viral shape (see figure 2.73). In the bacteriophage complex form, the genome is located within the polyhedral head and the sheath connects the head to the tail fibers and tail pins that help the virus attach to receptors on the host cell’s surface. Poxviruses that have complex shapes are often brick shaped, with intricate surface characteristics not seen in the other categories of capsid.
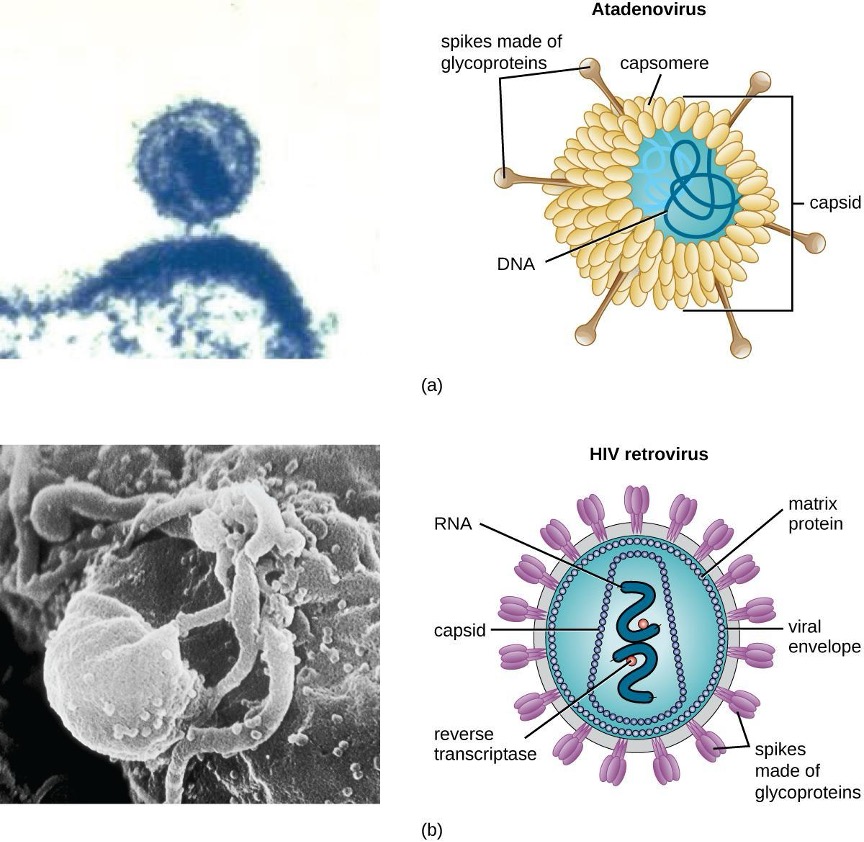
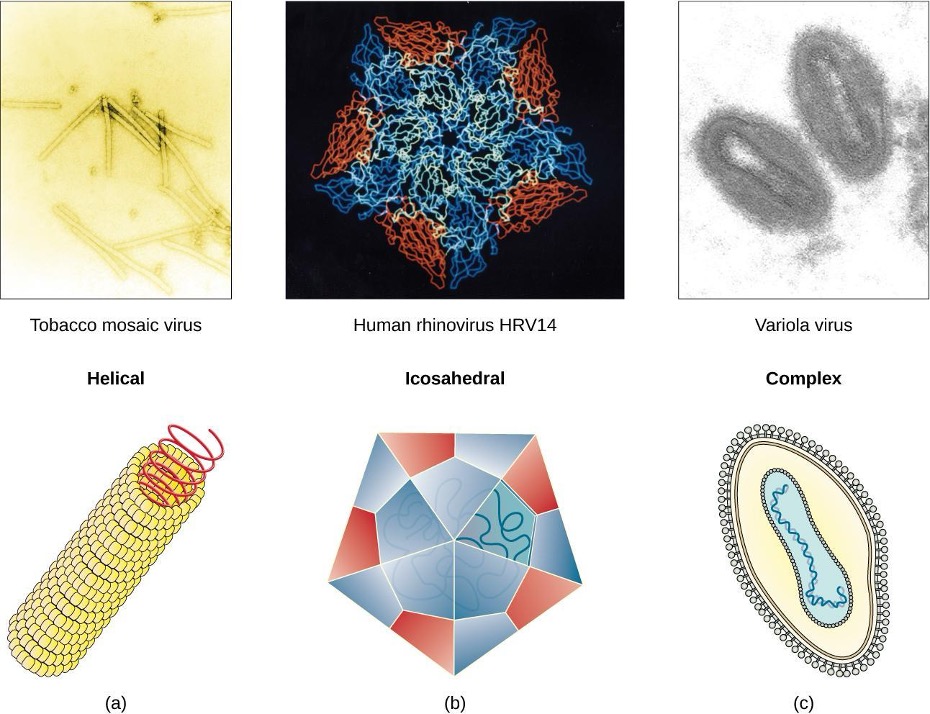
Classification and Taxonomy of Viruses
To date, the ICTV has classified known viruses in seven orders, 96 families, and 350 genera. Viral family names end in –viridae (e.g, Parvoviridae) and genus names end in −virus (e.g., Parvovirus). The names of viral orders, families, and genera are all italicized. When referring to a viral species, we often use a genus and species epithet such as Pandoravirus dulcis or Pandoravirus salinus.
The Baltimore classification system is an alternative to ICTV nomenclature. The Baltimore system classifies viruses according to their genomes (DNA or RNA, single versus double stranded, and mode of replication). This system thus creates seven groups of viruses that have common genetics and biology.
Aside from formal systems of nomenclature, viruses are often informally grouped into categories based on chemistry, morphology, or other characteristics they share in common. Categories may include naked or enveloped structure, single-stranded (ss) or double-stranded (ds) DNA or ss or ds RNA genomes, segmented or non-segmented genomes, and positive-strand (+) or negative-strand (−) RNA. For example, herpes viruses can be classified as a dsDNA enveloped virus; human immunodeficiency virus (HIV) is a +ssRNA enveloped virus, and tobacco mosaic virus is a +ssRNA virus. Other characteristics such as host specificity, tissue specificity, capsid shape, and special genes or enzymes may also be used to describe groups of similar viruses. Table 2.19 lists some of the most common viruses that are human pathogens by genome type.
Genome | Family | Example Virus | Clinical Features |
---|---|---|---|
dsDNA, enveloped | Poxviridae | Orthopoxvirus | Skin papules, pustules, lesions |
Poxviridae | Parapoxvirus | Skin lesions | |
Herpesviridae | Simplexvirus | Cold sores, genital herpes, sexually transmitted disease | |
dsDNA, naked | Adenoviridae | Atadenovirus | Respiratory infection (common cold) |
Papillomaviridae | Papillomavirus | Genital warts, common, and plantar warts cervical, vaginal or oropharyngeal cancer | |
Reoviridae | Reovirus | Gastroenteritis severe diarrhea (stomach flu) | |
ssDNA, naked | Parvoviridae | Adeno-associated dependoparvovirus A | Respiratory tract infection |
Parvoviridae | Adeno-associated dependoparvovirus B | Respiratory tract infection | |
dsRNA, naked | Reoviridae | Rotavirus | Gastroenteritis |
+ssRNA, naked | Picornaviridae | Enterovirus C | Poliomyelitis |
Picornaviridae | Rhinovirus | Upper respiratory tract infection (common cold) | |
Picornaviridae | Hepatovirus | Hepatitis | |
+ssRNA, enveloped | Togaviridae | Alphavirus | Encephalitis, hemorrhagic fever |
Togaviridae | Rubivirus | Rubella | |
Retroviridae | Lentivirus | Acquired immune deficiency syndrome (AIDS) | |
−ssRNA, enveloped | Filoviridae | Zaire Ebolavirus | Hemorrhagic fever |
Orthomyxoviridae | Influenzavirus A, B, C | Flu | |
Rhabdoviridae | Lyssavirus | Rabies |
Table 2.19: Common pathogenic viruses
2.11 The Viral Life Cycle
All viruses depend on cells for reproduction and metabolic processes. By themselves, viruses do not encode for all of the enzymes necessary for viral replication. But within a host cell, a virus can commandeer cellular machinery to produce more viral particles. Bacteriophages replicate only in the cytoplasm, since prokaryotic cells do not have a nucleus or organelles. In eukaryotic cells, most DNA viruses can replicate inside the nucleus, with an exception observed in the large DNA viruses, such as the poxviruses, that can replicate in the cytoplasm. With a few exceptions, RNA viruses that infect animal cells replicate in the cytoplasm. An important exception that will be highlighted later is Influenza virus.
The Life Cycle of Viruses with Prokaryote Hosts
The life cycle of bacteriophages has been a good model for understanding how viruses affect the cells they infect, since similar processes have been observed for eukaryotic viruses, which can cause immediate death of the cell or establish a latent or chronic infection. Virulent phages typically lead to the death of the cell through cell lysis. Temperate phages, on the other hand, can become part of a host chromosome and are replicated with the cell genome until such time as they are induced to make newly assembled viruses, or progeny viruses.
The Lytic Cycle
During the lytic cycle of virulent phage, the bacteriophage takes over the cell, reproduces new phages, and destroys the cell. T-even phage is a good example of a well-characterized class of virulent phages. There are five stages in the bacteriophage lytic cycle (see figure 2.74). Attachment is the first stage in the infection process in which the phage interacts with specific bacterial surface receptors (e.g., lipopolysaccharides and OmpC protein on host surfaces). Most phages have a narrow host range and may infect one species of bacteria or one strain within a species. This unique recognition can be exploited for targeted treatment of bacterial infection by phage therapy or for phage typing to identify unique bacterial subspecies or strains. The second stage of infection is entry or penetration. This occurs through contraction of the tail sheath, which acts like a hypodermic needle to inject the viral genome through the cell wall and membrane. The phage head and remaining components remain outside the bacteria.
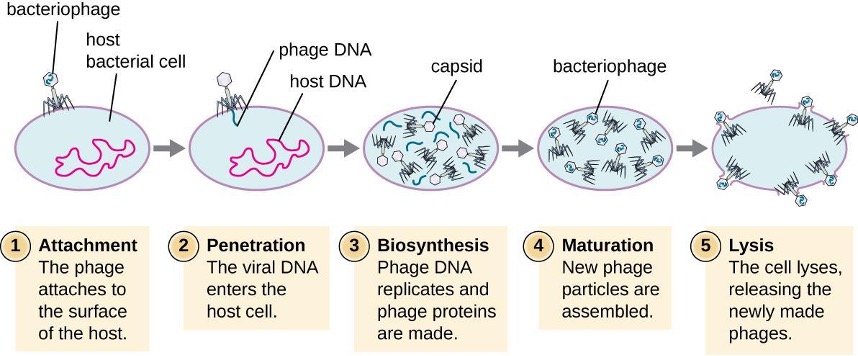
The third stage of infection is biosynthesis of new viral components. After entering the host cell, the virus synthesizes virus-encoded endonucleases to degrade the bacterial chromosome. It then hijacks the host cell to replicate, transcribe, and translate the necessary viral components (capsomeres, sheath, base plates, tail fibers, and viral enzymes) for the assembly of new viruses. Polymerase genes are usually expressed early in the cycle, while capsid and tail proteins are expressed later. During the maturation phase, new virions are created. To liberate free phages, the bacterial cell wall is disrupted by phage proteins such as holin or lysozyme. The final stage is the release of the newly made phages. Mature viruses burst out of the host cell in a process called lysis and the progeny viruses are liberated into the environment to infect new cells.
The Lysogenic Cycle
In a lysogenic cycle, the phage genome also enters the cell through attachment and penetration. A prime example of a phage with this type of life cycle is the lambda phage. During the lysogenic cycle, instead of killing the host, the phage genome integrates into the bacterial chromosome and becomes part of the host. The integrated phage genome is called a prophage. A bacterial host with a prophage is called a lysogen. The process in which a bacterium is infected by a temperate phage is called lysogeny. It is typical of temperate phages to be latent or inactive within the cell. As the bacterium replicates its chromosome, it also replicates the phage’s DNA and passes it on to new daughter cells during reproduction. The presence of the phage may alter the phenotype of the bacterium, since it can bring in extra genes (e.g., toxin genes that can increase bacterial virulence). This change in the host phenotype is called lysogenic conversion or phage conversion. Some bacteria, such as Vibrio cholerae and Clostridium botulinum, are less virulent in the absence of the prophage. The phages infecting these bacteria carry the toxin genes in their genome and enhance the virulence of the host when the toxin genes are expressed. In the case of V. cholera, phage-encoded toxins can cause severe diarrhea; in C. botulinum, the toxin can cause paralysis. During lysogeny, the prophage will persist in the host chromosome until induction, which results in the excision of the viral genome from the host chromosome. After induction has occurred the temperate phage can proceed through a lytic cycle and then undergo lysogeny in a newly infected cell (see figure 2.75).
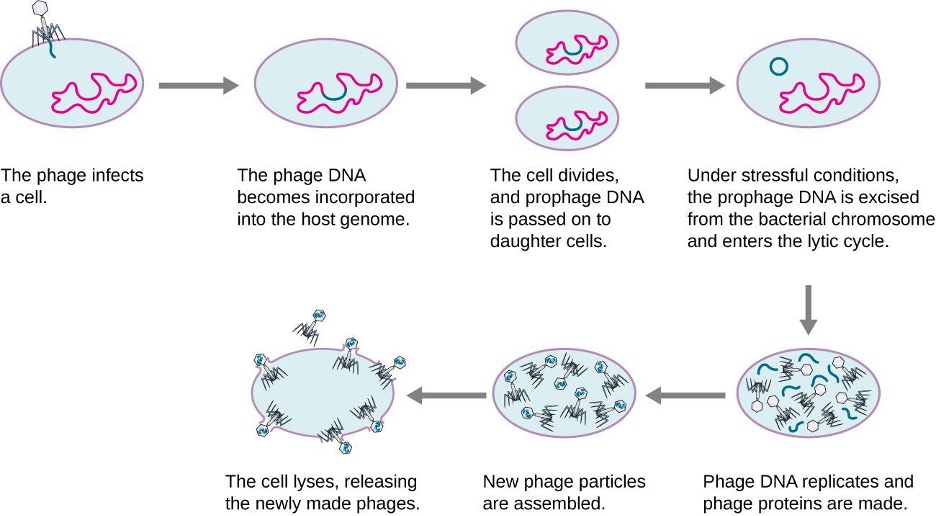
Transduction
Transduction occurs when a bacteriophage transfers bacterial DNA from one bacterium to another during sequential infections. There are two types of transduction: generalized and specialized transduction. During the lytic cycle of viral replication, the virus hijacks the host cell, degrades the host chromosome, and makes more viral genomes. As it assembles and packages DNA into the phage head, packaging occasionally makes a mistake. Instead of packaging viral DNA, it takes a random piece of host DNA and inserts it into the capsid. Once released, this virion will then inject the former host’s DNA into a newly infected host. The asexual transfer of genetic information can allow for DNA recombination to occur, thus providing the new host with new genes (e.g., an antibiotic-resistance gene, or a sugar-metabolizing gene). Generalized transduction occurs when a random piece of bacterial chromosomal DNA is transferred by the phage during the lytic cycle. Specialized transduction occurs at the end of the lysogenic cycle, when the prophage is excised and the bacteriophage enters the lytic cycle. Since the phage is integrated into the host genome, the prophage can replicate as part of the host. However, some conditions (e.g., ultraviolet light exposure or chemical exposure) stimulate the prophage to undergo induction, causing the phage to excise from the genome, enter the lytic cycle, and produce new phages to leave host cells. During the process of excision from the host chromosome, a phage may occasionally remove some bacterial DNA near the site of viral integration. The phage and host DNA from one or both ends of the integration site are packaged within the capsid. From here they are transferred to the new, infected host. Since the DNA transferred by the phage is not randomly packaged but is instead a specific piece of DNA near the site of integration, this mechanism of gene transfer is referred to as specialized transduction (see figure 2.76). The DNA can then recombine with the host chromosome, giving the latter new characteristics. Transduction seems to play an important role in the evolutionary process of bacteria, giving them a mechanism for asexual exchange of genetic information.
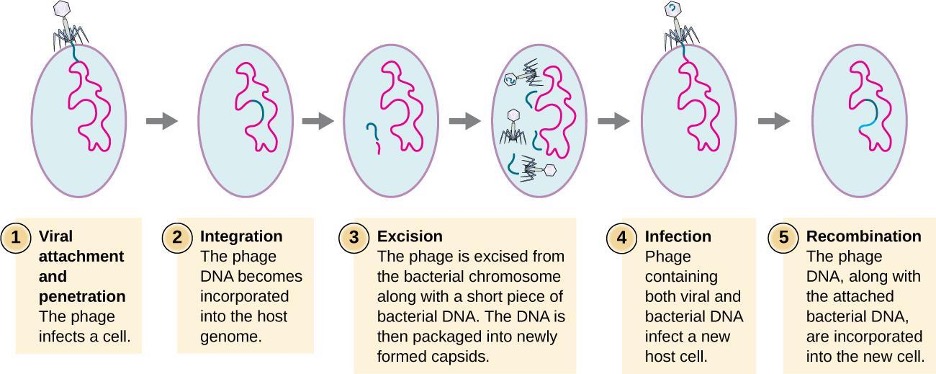
Life Cycle of Viruses with Animal Hosts
Lytic animal viruses follow similar infection stages to bacteriophages: attachment, penetration, biosynthesis, maturation, and release (see figure 2.77). However, the mechanisms of penetration, nucleic-acid biosynthesis, and release differ between bacterial and animal viruses. After binding to host receptors, animal viruses enter through endocytosis (engulfment by the host cell) or through membrane fusion (viral envelope with the host cell membrane). Many viruses are host specific, meaning they only infect a certain type of host; and most viruses only infect certain types of cells within tissues. This specificity is called a tissue tropism. Examples of this are demonstrated by the poliovirus, which exhibits tropism for the tissues of the brain and spinal cord, or the influenza virus, which has a primary tropism for the respiratory tract.
Animal viruses do not always express their genes using the normal flow of genetic information—from DNA to RNA to protein. Some viruses have a dsDNA genome like cellular organisms and can follow the normal flow. However, others may have ssDNA, dsRNA, or ssRNA genomes. The nature of the genome determines how the genome is replicated and expressed as viral proteins. If a genome is ssDNA, host enzymes will be used to synthesize a second strand that is complementary to the genome strand, thus producing dsDNA. The dsDNA can now be replicated, transcribed, and translated similar to host DNA.
If the viral genome is RNA, a different mechanism must be used. There are three types of RNA genome: dsRNA, positive (+) single-strand (+ssRNA) or negative (−) single-strand RNA (−ssRNA). If a virus has a +ssRNA genome, it can be translated directly to make viral proteins. Viral genomic +ssRNA acts like cellular mRNA. However, if a virus contains a −ssRNA genome, the host ribosomes cannot translate it until the −ssRNA is replicated into +ssRNA by viral RNA-dependent RNA polymerase (RdRP) (see figure 2.78). The RdRP is brought in by the virus and can be used to make +ssRNA from the original −ssRNA genome. The RdRP is also an important enzyme for the replication of dsRNA viruses, because it uses the negative strand of the double-stranded genome as a template to create +ssRNA. The newly synthesized +ssRNA copies can then be translated by cellular ribosomes.
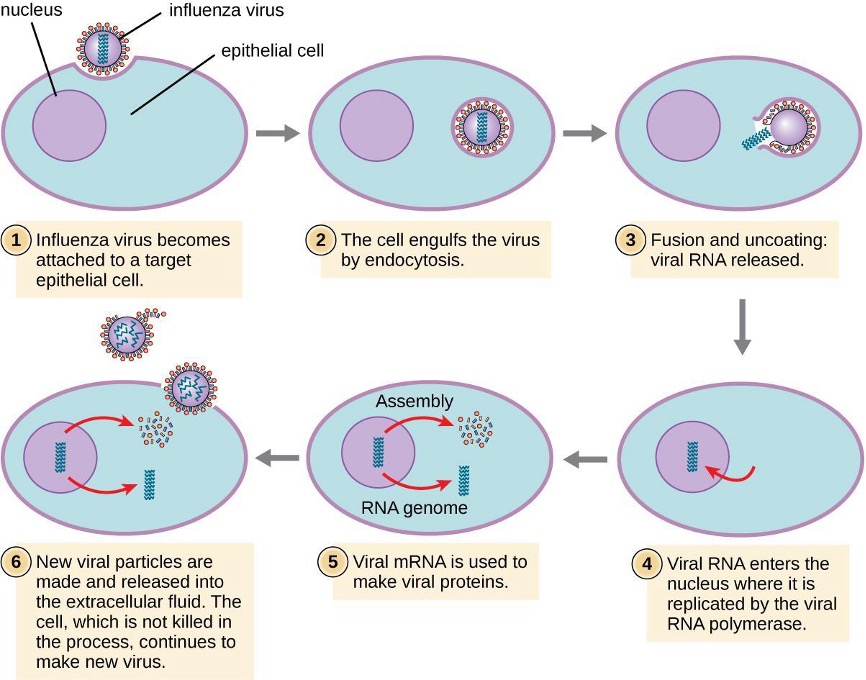
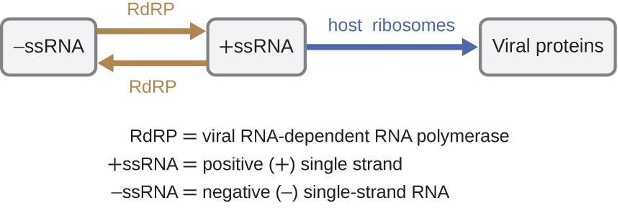
An alternative mechanism for viral nucleic acid synthesis is observed in the retroviruses, which are +ssRNA viruses (see figure 2.79). Single-stranded RNA viruses such as HIV carry a special enzyme called reverse transcriptase within the capsid that synthesizes a complementary ssDNA (cDNA) copy using the +ssRNA genome as a template. The ssDNA is then made into dsDNA, which can integrate into the host chromosome and become a permanent part of the host. The integrated viral genome is called a provirus. The virus can now remain in the host for a long time to establish a chronic infection. The provirus stage is similar to the prophage stage in a bacterial infection during the lysogenic cycle. However, unlike prophage, the provirus does not undergo excision after splicing into the genome.
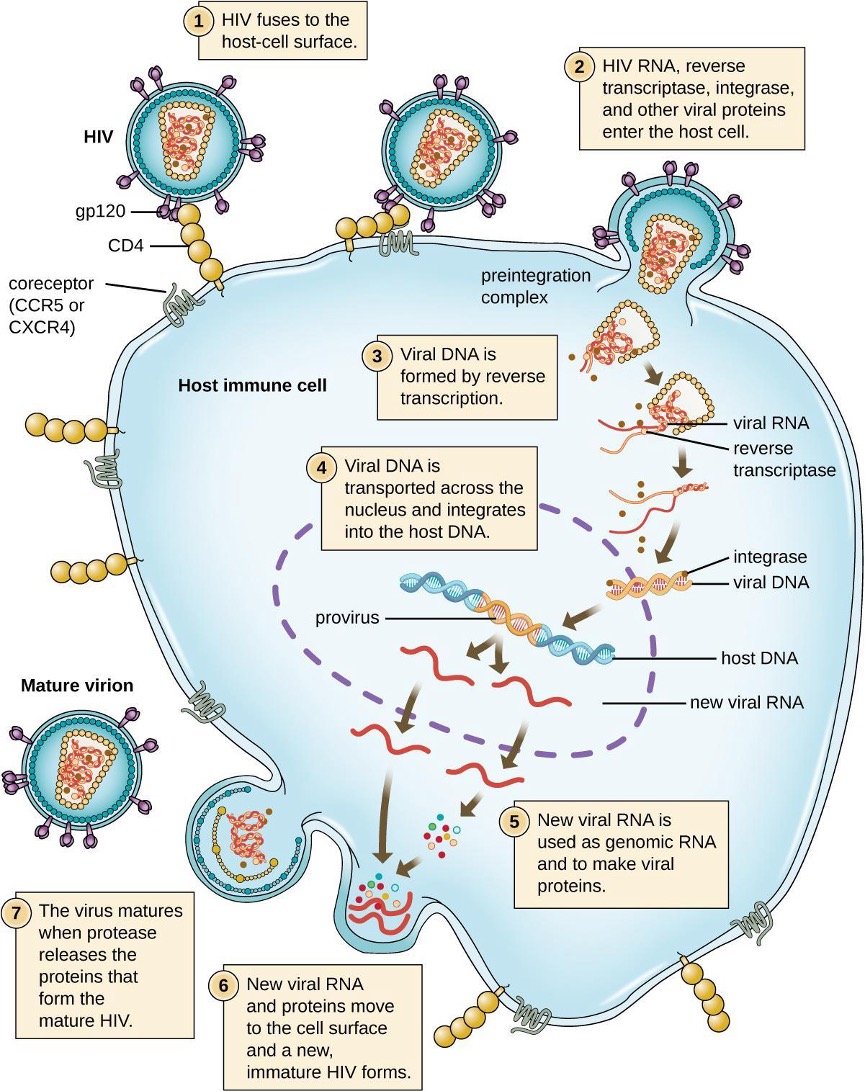
Persistent Infections
Persistent infection occurs when a virus is not completely cleared from the system of the host but stays in certain tissues or organs of the infected person. The virus may remain silent or undergo productive infection without seriously harming or killing the host. Mechanisms of persistent infection may involve the regulation of the viral or host gene expressions or the alteration of the host immune response. The two primary categories of persistent infections are latent infection and chronic infection. Examples of viruses that cause latent infections include herpes simplex virus (oral and genital herpes), varicella-zoster virus (chickenpox and shingles), and Epstein-Barr virus (mononucleosis). Hepatitis C virus and HIV are two examples of viruses that cause long-term chronic infections.
Latent Infection
Not all animal viruses undergo replication by the lytic cycle. There are viruses that are capable of remaining hidden or dormant inside the cell in a process called latency. These types of viruses are known as latent viruses and may cause latent infections. In some cases, viruses capable of latency may initially cause an acute infection before becoming dormant.
For example, the varicella-zoster virus infects many cells throughout the body and causes chickenpox, characterized by a rash of blisters covering the skin. About 10 to 12 days post infection, the disease resolves and the virus goes dormant, living within nerve-cell ganglia for years. During this time, the virus does not kill the nerve cells or continue replicating. It is not clear why the virus stops replicating within the nerve cells and expresses few viral proteins but, in some cases, typically after many years of dormancy, the virus is reactivated and causes a new disease called shingles (figure 2.80). Whereas chickenpox affects many areas throughout the body, shingles is a nerve cell-specific disease emerging from the ganglia in which the virus was dormant.
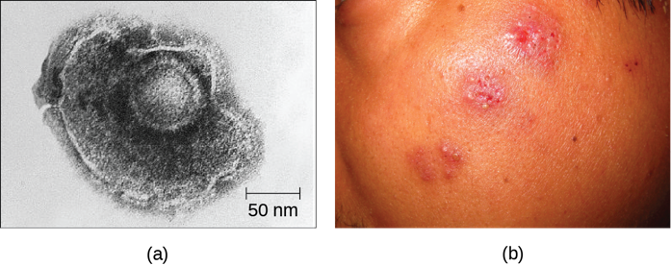
Latent viruses may remain dormant by existing as circular viral genome molecules outside of the host chromosome. Others become proviruses by integrating into the host genome. During dormancy, viruses do not cause any symptoms of disease and may be difficult to detect. A patient may be unaware that he or she is carrying the virus unless a viral diagnostic test has been performed.
Chronic Infection
A chronic infection is a disease with symptoms that are recurrent or persistent over a long time. Some viral infections can be chronic if the body is unable to eliminate the virus. HIV is an example of a virus that produces a chronic infection, often after a long period of latency. Once a person becomes infected with HIV, the virus can be detected in tissues continuously thereafter, but untreated patients often experience no symptoms for years. However, the virus maintains chronic persistence through several mechanisms that interfere with immune function, including preventing expression of viral antigens on the surface of infected cells, altering immune cells themselves, restricting expression of viral genes, and rapidly changing viral antigens through mutation. Eventually, the damage to the immune system results in progression of the disease leading to acquired immunodeficiency syndrome (AIDS). The various mechanisms that HIV uses to avoid being cleared by the immune system are also used by other chronically infecting viruses, including the hepatitis C virus.
Viral Growth Curve
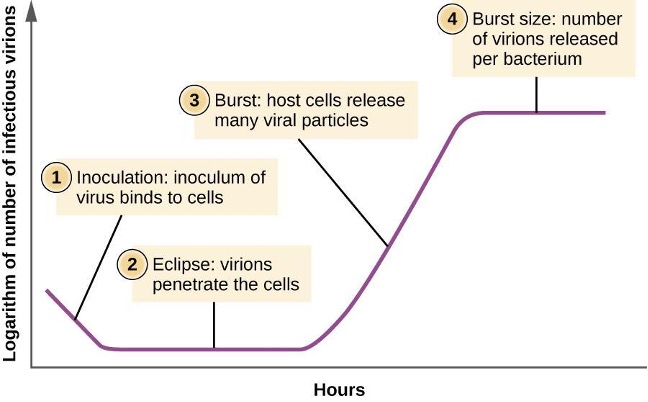
Unlike the growth curve for a bacterial population, the growth curve for a virus population over its life cycle does not follow a sigmoidal curve. During the initial stage, an inoculum of virus causes infection. In the eclipse phase, viruses bind and penetrate the cells with no virions detected in the medium. The chief difference that next appears in the viral growth curve compared to a bacterial growth curve occurs when virions are released from the lysed host cell at the same time. Such an occurrence is called a burst, and the number of virions per bacterium released is described as the burst size. In a one-step multiplication curve for bacteriophage, the host cells lyse, releasing many viral particles to the medium, which leads to a very steep rise in viral titer (the number of virions per unit volume). If no viable host cells remain, the viral particles begin to degrade during the decline of the culture (see figure 2.81).
Detection of a Virus
Regardless of the method of cultivation, once a virus has been introduced into a whole host organism, embryo, or tissue-culture cell, a sample can be prepared from the infected host, embryo, or cell line for further analysis under a brightfield, electron, or fluorescent microscope. Cytopathic effects (CPEs) are distinct observable cell abnormalities due to viral infection. CPEs can include loss of adherence to the surface of the container, changes in cell shape from flat to round, shrinkage of the nucleus, vacuoles in the cytoplasm, fusion of cytoplasmic membranes and the formation of multinucleated syncytia, inclusion bodies in the nucleus or cytoplasm, and complete cell lysis (see table 2.20).
Further pathological changes include viral disruption of the host genome and altering normal cells into transformed cells, which are the types of cells associated with carcinomas and sarcomas. The type or severity of the CPE depends on the type of virus involved. Table 2.20 lists CPEs for specific viruses.
Virus | Cytopathic Effect | Example |
---|---|---|
Paramyxovirus | Syncytium and faint basophilic cytoplasmic inclusion bodies | ![]() |
Poxvirus | Pink eosinophilic cytoplasmic inclusion bodies (arrows) and cell swelling | ![]() |
Herpesvirus | Cytoplasmic stranding (arrow) and nuclear inclusion bodies (dashed arrow) | ![]() |
Adenovirus | Cell enlargement, rounding, and distinctive "grape-like" clusters | ![]() |
Table 2.20: Cytopathic effects of specific viruses
Hemagglutination Assay
A serological assay is used to detect the presence of certain types of viruses in patient serum. Serum can be used in a direct assay called a hemagglutination assay to detect specific types of viruses in the patient’s sample. Hemagglutination is the agglutination (clumping) together of erythrocytes (red blood cells). Many viruses produce surface proteins or spikes called hemagglutinins that can bind to receptors on the membranes of erythrocytes and cause the cells to agglutinate. Hemagglutination is observable without using the microscope, but this method does not always differentiate between infectious and noninfectious viral particles, since both can agglutinate erythrocytes.
To identify a specific pathogenic virus using hemagglutination, we must use an indirect approach. Proteins called antibodies, generated by the patient’s immune system to fight a specific virus, can be used to bind to components such as hemagglutinins that are uniquely associated with specific types of viruses. The binding of the antibodies with the hemagglutinins found on the virus subsequently prevent erythrocytes from directly interacting with the virus. So when erythrocytes are added to the antibody-coated viruses, there is no appearance of agglutination; agglutination has been inhibited. We call these types of indirect assays for virus-specific antibodies hemagglutination inhibition (HAI) assays. HAI can be used to detect the presence of antibodies specific to many types of viruses that may be causing or have caused an infection in a patient even months or years after infection (see figure 2.82).
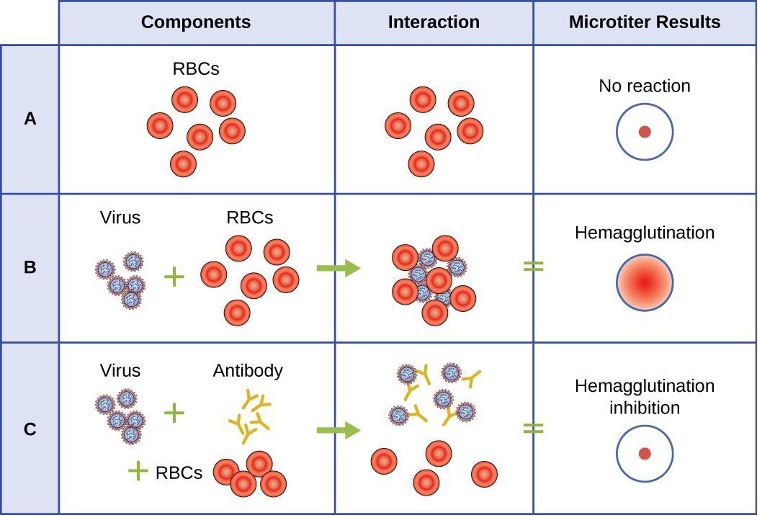
Nucleic Acid Amplification Test
Nucleic acid amplification tests (NAAT) are used in molecular biology to detect unique nucleic acid sequences of viruses in patient samples. Polymerase chain reaction (PCR) is an NAAT used to detect the presence of viral DNA in a patient’s tissue or body fluid sample. PCR is a technique that amplifies (i.e., synthesizes many copies) of a viral DNA segment of interest. Using PCR, short nucleotide sequences called primers bind to specific sequences of viral DNA, enabling identification of the virus.
Reverse transcriptase-PCR (RT-PCR) is an NAAT used to detect the presence of RNA viruses. RT-PCR differs from PCR in that the enzyme reverse transcriptase (RT) is used to make cDNA from the small amount of viral RNA in the specimen. The cDNA can then be amplified by PCR. Both PCR and RT-PCR are used to detect and confirm the presence of the viral nucleic acid in patient specimens.
Enzyme immunoassays
Enzyme immunoassays (EIAs) rely on the ability of antibodies to detect and attach to specific biomolecules called antigens. The detecting antibody attaches to the target antigen with a high degree of specificity in what might be a complex mixture of biomolecules. Also included in this type of assay is a colorless enzyme attached to the detecting antibody. The enzyme acts as a tag on the detecting antibody and can interact with a colorless substrate, leading to the production of a colored end product. EIAs often rely on layers of antibodies to capture and react with antigens, all of which are attached to a membrane filter (see figure 2.83). EIAs for viral antigens are often used as preliminary screening tests. If the results are positive, further confirmation will require tests with even greater sensitivity, such as a western blot or an NAAT.
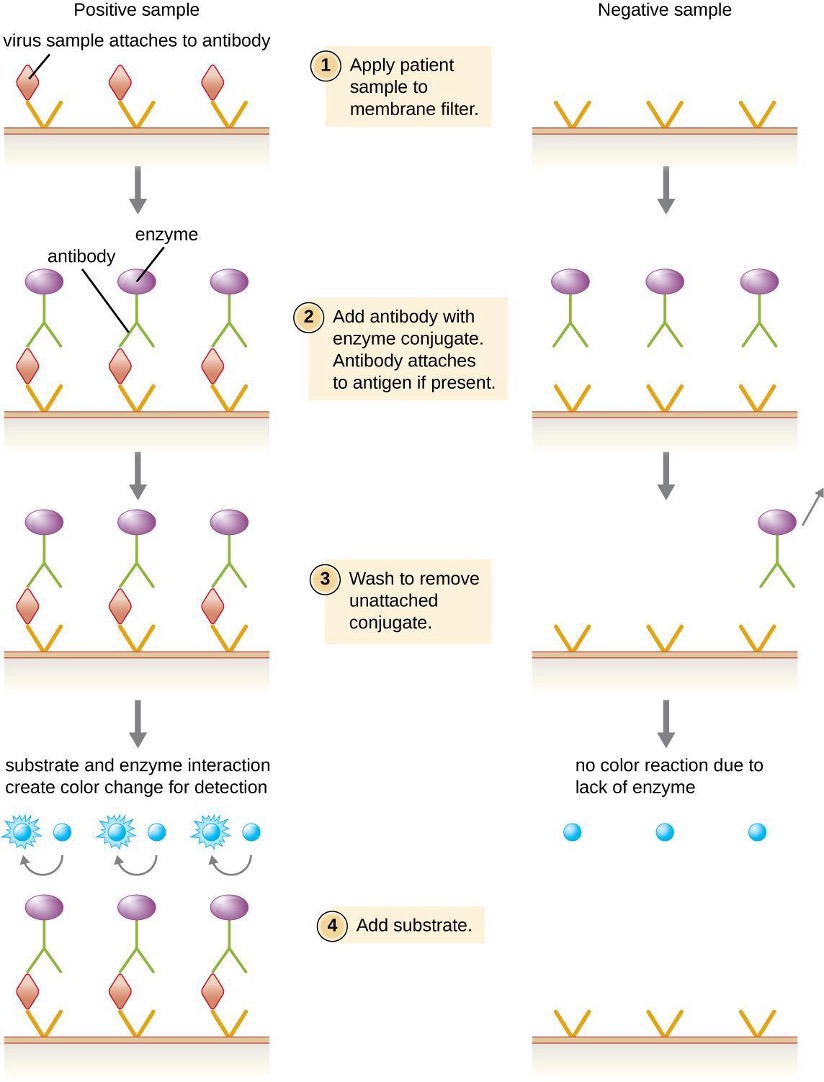
2.12 Viroids, Virusoids, and Prions
Research attempts to discover the causative agents of previously uninvestigated diseases have led to the discovery of nonliving disease agents quite different from viruses. These include particles consisting only of RNA or only of protein that, nonetheless, are able to self-propagate at the expense of a host—a key similarity to viruses that allows them to cause disease conditions. To date, these discoveries include viroids, virusoids, and the proteinaceous prions.
Prions
At one time, scientists believed that any infectious particle must contain DNA or RNA. Then, in 1982, Stanley Prusiner, a medical doctor studying scrapie (a fatal, degenerative disease in sheep) discovered that the disease was caused by proteinaceous infectious particles, or prions. Because proteins are acellular and do not contain DNA or RNA, Prusiner’s findings were originally met with resistance and skepticism; however, his research was eventually validated, and he received the Nobel Prize in Physiology or Medicine in 1997.
A prion is a misfolded rogue form of a normal protein (PrPc) found in the cell. This rogue prion protein (PrPsc), which may be caused by a genetic mutation or occur spontaneously, can be infectious, stimulating other endogenous normal proteins to become misfolded, forming plaques (see figure 2.84). Today, prions are known to cause various forms of transmissible spongiform encephalopathy (TSE) in humans and animals. TSE is a rare degenerative disorder that affects the brain and nervous system. The accumulation of rogue proteins causes the brain tissue to become sponge-like, killing brain cells and forming holes in the tissue, leading to brain damage, loss of motor coordination, and dementia (see figure 2.85). Infected individuals are mentally impaired and become unable to move or speak. There is no cure, and the disease progresses rapidly, eventually leading to death within a few months or years.
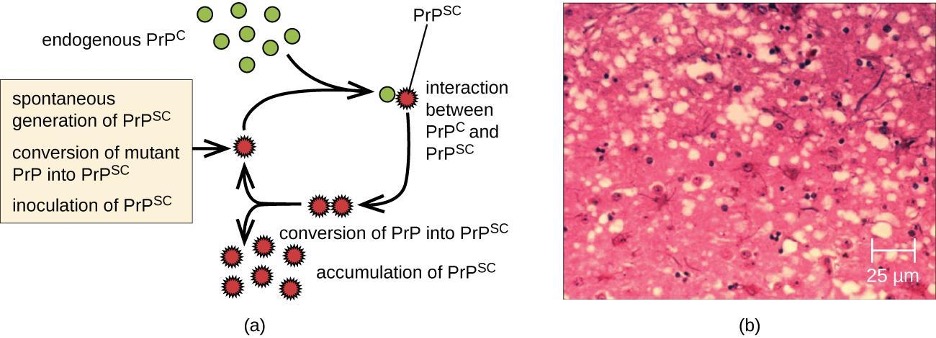
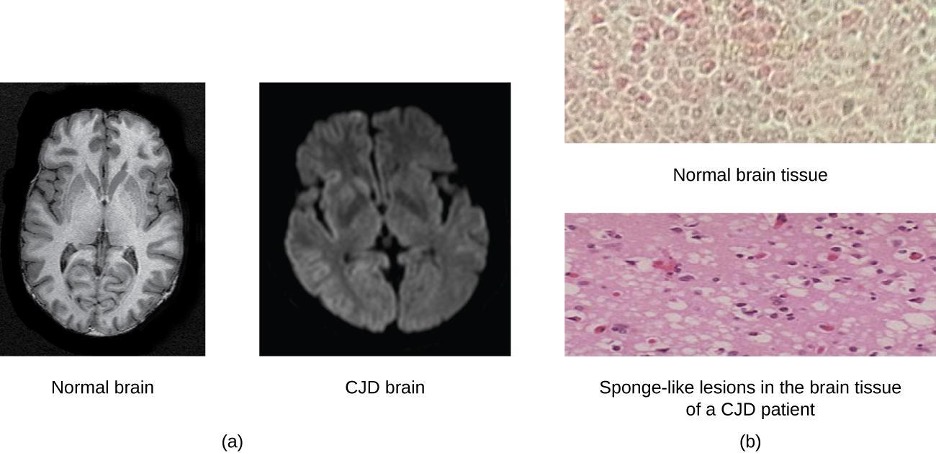
TSEs in humans include kuru, fatal familial insomnia, Gerstmann-Straussler-Scheinker disease, and Creutzfeldt-Jakob disease (see figure 2.85). TSEs in animals include mad cow disease, scrapie (in sheep and goats), and chronic wasting disease (in elk and deer). TSEs can be transmitted between animals and from animals to humans by eating contaminated meat or animal feed. Transmission between humans can occur through heredity (as is often the case with GSS and CJD) or by contact with contaminated tissue, as might occur during a blood transfusion or organ transplant. There is no evidence for transmission via casual contact with an infected person. Table 2.21 lists TSEs that affect humans and their modes of transmission.
Disease | Mechanism(s) of Transmission* |
---|---|
Sporadic CJD (sCJD) | Not known; possibly by alteration of normal prior protein (PrP) to rogue form due to somatic mutation |
Variant CJD (vCJD) | Eating contaminated cattle products and by secondary bloodborne transmission |
Familial CJD (fCJD) | Mutation in germline PrP gene |
Iatrogenic CJD (iCJD) | Contaminated neurosurgical instruments, corneal graft, gonadotrophic hormone, and, secondarily, by blood transfusion |
Kuru | Eating infected meat through ritualistic cannibalism |
Gerstmann-Straussler-Scheinker disease (GSS) | Mutation in germline PrP gene |
Fatal familial insomnia (FFI) | Mutation in germline PrP gene |
Table 2.21: Transmissible spongiform encephalopathies (TSEs) in humans. *National Institute of Neurological Disorders and Stroke. “Creutzfeldt-Jakob Disease Fact Sheet.” http://www.ninds.nih.gov/disorders/cjd/detail_cjd.htm (accessed December 31, 2015).
Prions are extremely difficult to destroy because they are resistant to heat, chemicals, and radiation. Even standard sterilization procedures do not ensure the destruction of these particles. Currently, there is no treatment or cure for TSE disease, and contaminated meats or infected animals must be handled according to federal guidelines to prevent transmission.
2.13 How Pathogens Cause Disease
For most infectious diseases, the ability to accurately identify the causative pathogen is a critical step in finding or prescribing effective treatments. Today’s physicians, patients, and researchers owe a sizable debt to the physician Robert Koch (1843–1910), who devised a systematic approach for confirming causative relationships between diseases and specific pathogens.
Koch’s Postulates
In 1884, Koch published four postulates (table 2.22) that summarized his method for determining whether a particular microorganism was the cause of a particular disease. Each of Koch’s postulates represents a criterion that must be met before a disease can be positively linked with a pathogen. In order to determine whether the criteria are met, tests are performed on laboratory animals and cultures from healthy and diseased animals are compared (figure 2.86).
Koch’s Postulates |
---|
(1) The suspected pathogen must be found in every case of disease and not be found in healthy individuals. |
(2) The suspected pathogen can be isolated and grown in pure culture. |
(3) A healthy test subject infected with the suspected pathogen must develop the same signs and symptoms of disease as seen in postulate 1. |
(4) The pathogen must be re-isolated from the new host and must be identical to the pathogen from postulate 2. |
Table 2.22: Koch’s postulates
In many ways, Koch’s postulates are still central to our current understanding of the causes of disease. However, advances in microbiology have revealed some important limitations in Koch’s criteria. Koch made several assumptions that we now know are untrue in many cases. The first relates to postulate 1, which assumes that pathogens are only found in diseased, not healthy, individuals. This is not true for many pathogens.
Koch’s second faulty assumption was that all healthy test subjects are equally susceptible to disease. We now know that individuals are not equally susceptible to disease. Individuals are unique in terms of their microbiota and the state of their immune system at any given time. The makeup of the resident microbiota can influence an individual’s susceptibility to an infection. Members of the normal microbiota play an important role in immunity by inhibiting the growth of transient pathogens. In some cases, the microbiota may prevent a pathogen from establishing an infection; in others, it may not prevent an infection altogether but may influence the severity or type of signs and symptoms.
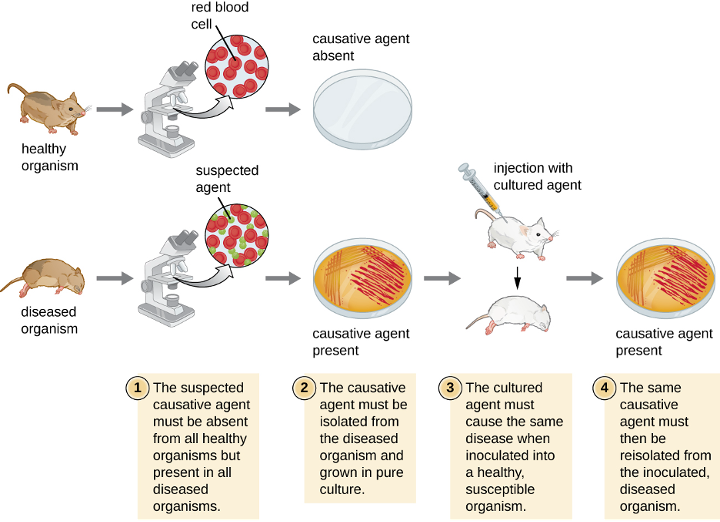
Koch also assumed that all pathogens are microorganisms that can be grown in pure culture (postulate 2) and that animals could serve as reliable models for human disease. However, we now know that not all pathogens can be grown in pure culture, and many human diseases cannot be reliably replicated in animal hosts. Viruses and certain bacteria, including Rickettsia and Chlamydia, are obligate intracellular pathogens that can grow only when inside a host cell. If a microbe cannot be cultured, a researcher cannot move past postulate 2. Likewise, without a suitable non-human host, a researcher cannot evaluate postulate 3 without deliberately infecting humans, which presents obvious ethical concerns. AIDS is an example of such a disease because the human immunodeficiency virus (HIV) only causes disease in humans.
Molecular Koch’s Postulates
In 1988, Stanley Falkow (1934–) proposed a revised form of Koch’s postulates known as molecular Koch’s postulates. These are listed in the left column of table 2.23. The premise for molecular Koch’s postulates is not in the ability to isolate a particular pathogen but rather to identify a gene that may cause the organism to be pathogenic.
Falkow’s modifications to Koch’s original postulates explain not only infections caused by intracellular pathogens but also the existence of pathogenic strains of organisms that are usually nonpathogenic. For example, the predominant form of the bacterium Escherichia coli is a member of the normal microbiota of the human intestine and is generally considered harmless. However, there are pathogenic strains of E. coli such as enterotoxigenic E. coli (ETEC) and enterohemorrhagic E. coli (O157:H7) (EHEC). We now know ETEC and EHEC exist because of the acquisition of new genes by the once-harmless E. coli, which, in the form of these pathogenic strains, is now capable of producing toxins and causing illness.
Molecular Koch’s Postulates | Application to EHEC |
---|---|
(1) The phenotype (sign or symptom of disease) should be associated only with pathogenic strains of a species. | EHEC causes intestinal inflammation and diarrhea, whereas nonpathogenic strains of E. coli do not. |
(2) Inactivation of the suspected gene(s) associated with pathogenicity should result in a measurable loss of pathogenicity. | One of the genes in EHEC encodes for Shiga toxin, a bacterial toxin (poison) that inhibits protein synthesis. Inactivating this gene reduces the bacteria’s ability to cause disease. |
(3) Reversion of the inactive gene should restore the disease phenotype. | By adding the gene that encodes the toxin back into the genome (e.g., with a phage or plasmid), EHEC’s ability to cause disease is restored. |
Table 2.23: Molecular Koch’s postulates applied to EHEC
Pathogenicity and Virulence
The ability of a microbial agent to cause disease is called pathogenicity, and the degree to which an organism is pathogenic is called virulence. Virulence is a continuum. On one end of the spectrum are organisms that are avirulent (not harmful) and on the other are organisms that are highly virulent. Highly virulent pathogens will almost always lead to a disease state when introduced to the body, and some may even cause multi-organ and body system failure in healthy individuals. Less virulent pathogens may cause an initial infection, but may not always cause severe illness. Pathogens with low virulence would more likely result in mild signs and symptoms of disease, such as low-grade fever, headache, or muscle aches. Some individuals might even be asymptomatic.
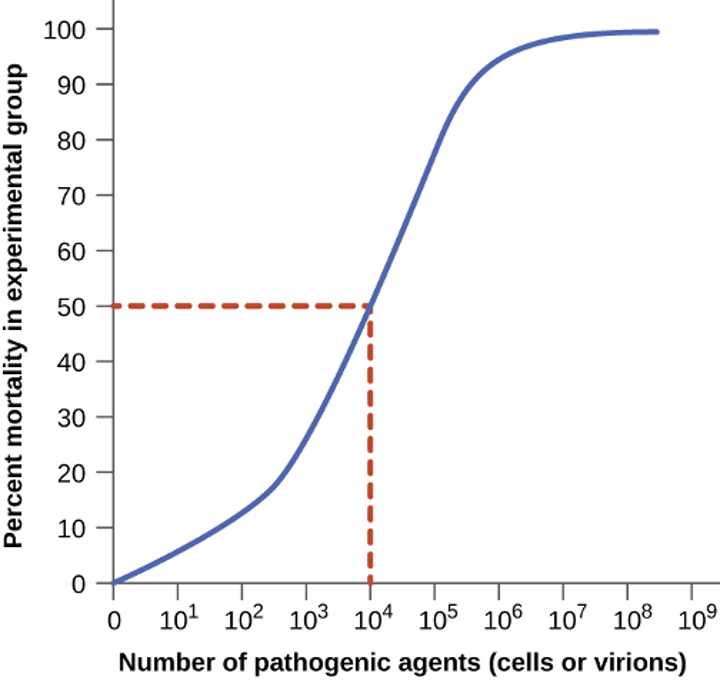
Virulence of a pathogen can be quantified using controlled experiments with laboratory animals. Two important indicators of virulence are the median infectious dose (ID50) and the median lethal dose (LD50), both of which are typically determined experimentally using animal models. The ID50 is the number of pathogen cells or virions required to cause active infection in 50% of inoculated animals. The LD50 is the number of pathogenic cells, virions, or amount of toxin required to kill 50% of infected animals. To calculate these values, each group of animals is inoculated with one of a range of known numbers of pathogen cells or virions. In graphs like the one shown in figure 2.87, the percentage of animals that have been infected (for ID50) or killed (for LD50) is plotted against the concentration of pathogen inoculated. Figure 2.87 represents data graphed from a hypothetical experiment measuring the LD50 of a pathogen. Interpretation of the data from this graph indicates that the LD50 of the pathogen for the test animals is 104 pathogen cells or virions (depending upon the pathogen studied).
Table 2.24 lists selected foodborne pathogens and their ID50 values in humans (as determined from epidemiologic data and studies on human volunteers). Keep in mind that these are median values. The actual infective dose for an individual can vary widely, depending on factors such as route of entry; the age, health, and immune status of the host; and environmental and pathogen-specific factors such as susceptibility to the acidic pH of the stomach. It is also important to note that a pathogen’s infective dose does not necessarily correlate with disease severity. For example, just a single cell of Salmonella enterica serotype Typhimurium can result in an active infection. The resultant disease, Salmonella gastroenteritis or salmonellosis, can cause nausea, vomiting, and diarrhea, but has a mortality rate of less than 1% in healthy adults. In contrast, S. enterica serotype Typhi has a much higher ID50, typically requiring as many as 1,000 cells to produce infection. However, this serotype causes typhoid fever, a much more systemic and severe disease that has a mortality rate as high as 10% in untreated individuals.
Pathogen | ID50 |
---|---|
Viruses | |
Hepatitis A virus | 10–100 |
Norovirus | 1–10 |
Rotavirus | 10–100 |
Bacteria | |
Escherichia coli, enterohemorrhagic (EHEC, serotype O157) | 10–100 |
E. coli, enteroinvasive (EIEC) | 200–5,000 |
E. coli, enteropathogenic (EPEC) | 10,000,000–10,000,000,000 |
E. coli, enterotoxigenic (ETEC) | 10,000,000–10,000,000,000 |
Salmonella enterica serovar Typhi | <1,000 |
S. enterica serovar Typhimurium | ≥1 |
Shigella dysenteriae | 10–200 |
Vibrio cholerae (serotypes O139, O1) | 1,000,000 |
V. parahemolyticus | 100,000,000 |
Protozoa | |
Giardia lamblia | 1 |
Cryptosporidium parvum | 10–100 |
Table 2.24: ID50 for selected foodborne diseases (credit: Food and Drug Administration. “Bad Bug Book, Foodborne Pathogenic Microorganisms and Natural Toxins.” 2nd ed. Silver Spring, MD: US Food and Drug Administration; 2012.)
Primary Pathogens versus Opportunistic Pathogens
Pathogens can be classified as either primary pathogens or opportunistic pathogens. A primary pathogen can cause disease in a host regardless of the host’s resident microbiota or immune system. An opportunistic pathogen, by contrast, can only cause disease in situations that compromise the host’s defenses, such as the body’s protective barriers, immune system, or normal microbiota. Individuals susceptible to opportunistic infections include the very young, the elderly, women who are pregnant, patients undergoing chemotherapy, people with immunodeficiencies (such as acquired immunodeficiency syndrome [AIDS]), patients who are recovering from surgery, and those who have had a breach of protective barriers (such as a severe wound or burn).
An example of a primary pathogen is enterohemorrhagic E. coli (EHEC), which produces a virulence factor known as Shiga toxin. This toxin inhibits protein synthesis, leading to severe and bloody diarrhea, inflammation, and renal failure, even in patients with healthy immune systems. Staphylococcus epidermidis, on the other hand, is an opportunistic pathogen that is among the most frequent causes of nosocomial disease.[22] S. epidermidis is a member of the normal microbiota of the skin, where it is generally avirulent. However, in hospitals, it can also grow in biofilms that form on catheters, implants, or other devices that are inserted into the body during surgical procedures. Once inside the body, S. epidermidis can cause serious infections such as endocarditis, and it produces virulence factors that promote the persistence of such infections.
Other members of the normal microbiota can also cause opportunistic infections under certain conditions. This often occurs when microbes that reside harmlessly in one body location end up in a different body system, where they cause disease. For example, E. coli normally found in the large intestine can cause a urinary tract infection if it enters the bladder. This is the leading cause of urinary tract infections among women.
Members of the normal microbiota may also cause disease when a shift in the environment of the body leads to overgrowth of a particular microorganism. For example, the yeast Candida is part of the normal microbiota of the skin, mouth, intestine, and vagina, but its population is kept in check by other organisms of the microbiota. If an individual is taking antibacterial medications, however, bacteria that would normally inhibit the growth of Candida can be killed off, leading to a sudden growth in the population of Candida, which is not affected by antibacterial medications because it is a fungus. An overgrowth of Candida can manifest as oral thrush (growth on mouth, throat, and tongue), a vaginal yeast infection, or cutaneous candidiasis. Other scenarios can also provide opportunities for Candida infections. Untreated diabetes can result in a high concentration of glucose in the saliva, which provides an optimal environment for the growth of Candida, resulting in thrush. Immunodeficiencies such as those seen in patients with HIV, AIDS, and cancer also lead to higher incidence of thrush. Vaginal yeast infections can result from decreases in estrogen levels during the menstruation or menopause. The amount of glycogen available to lactobacilli in the vagina is controlled by levels of estrogen; when estrogen levels are low, lactobacilli produce less lactic acid. The resultant increase in vaginal pH allows overgrowth of Candida in the vagina.
Stages of Pathogenesis
To cause disease, a pathogen must successfully achieve four steps or stages of pathogenesis: exposure (contact), adhesion (colonization), invasion, and infection. The pathogen must be able to gain entry to the host, travel to the location where it can establish an infection, evade or overcome the host’s immune response, and cause damage (i.e., disease) to the host. In many cases, the cycle is completed when the pathogen exits the host and is transmitted to a new host.
Exposure
An encounter with a potential pathogen is known as exposure or contact. The food we eat and the objects we handle are all ways that we can come into contact with potential pathogens. Yet, not all contacts result in infection and disease. For a pathogen to cause disease, it needs to be able to gain access into host tissue. An anatomic site through which pathogens can pass into host tissue is called a portal of entry. These are locations where the host cells are in direct contact with the external environment. Major portals of entry are identified in figure 2.88 and include the skin, mucous membranes, and parenteral routes.
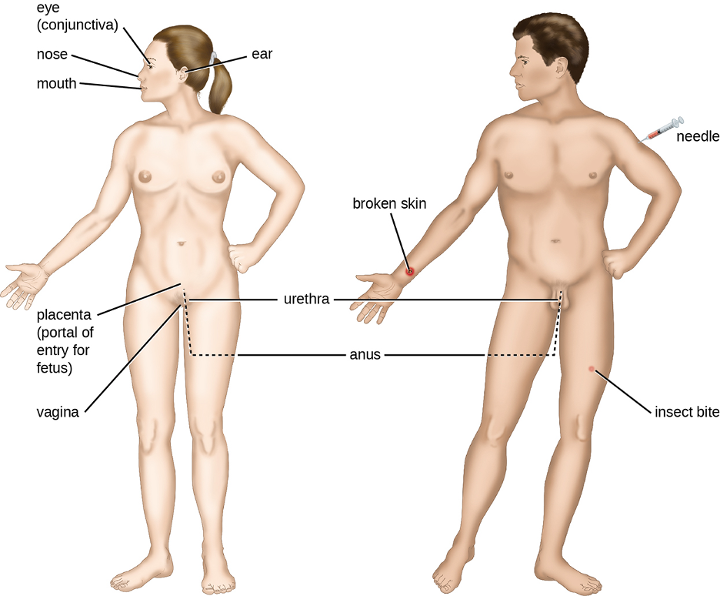
Mucosal surfaces are the most important portals of entry for microbes; these include the mucous membranes of the respiratory tract, the gastrointestinal tract, and the genitourinary tract. Although most mucosal surfaces are in the interior of the body, some are contiguous with the external skin at various body openings, including the eyes, nose, mouth, urethra, and anus.
Most pathogens are suited to a particular portal of entry. A pathogen’s portal specificity is determined by the organism’s environmental adaptations and by the enzymes and toxins they secrete. The respiratory and gastrointestinal tracts are particularly vulnerable portals of entry because particles that include microorganisms are constantly inhaled or ingested, respectively.
Pathogens can also enter through a breach in the protective barriers of the skin and mucous membranes. Pathogens that enter the body in this way are said to enter by the parenteral route. For example, the skin is a good natural barrier to pathogens, but breaks in the skin (e.g., wounds, insect bites, animal bites, needle pricks) can provide a parenteral portal of entry for microorganisms.
In pregnant women, the placenta normally prevents microorganisms from passing from the mother to the fetus. However, a few pathogens are capable of crossing the blood-placental barrier. The gram-positive bacterium Listeria monocytogenes, which causes the foodborne disease listeriosis, is one example that poses a serious risk to the fetus and can sometimes lead to spontaneous abortion. Other pathogens that can pass the placental barrier to infect the fetus are known collectively by the acronym TORCH (table 2.25).
Transmission of infectious diseases from mother to baby is also a concern at the time of birth when the baby passes through the birth canal. Babies whose mothers have active chlamydia or gonorrhea infections may be exposed to the causative pathogens in the vagina, which can result in eye infections that lead to blindness. To prevent this, it is standard practice to administer antibiotic drops to infants’ eyes shortly after birth.
Disease | Pathogen | |
---|---|---|
T | Toxoplasmosis | Toxoplasma gondii (protozoan) |
O* | Syphilis Chickenpox Hepatitis B HIV Fifth disease (erythema infectiosum) |
Treponema pallidum (bacterium) Varicella-zoster virus (human herpesvirus 3) Hepatitis B virus (hepadnavirus) Retrovirus Parvovirus B19 |
R | Rubella (German measles) | Togavirus |
C | Cytomegalovirus | Human herpesvirus 5 |
H | Herpes | Herpes simplex viruses (HSV) 1 and 2 |
*The O in TORCH stands for "other." |
Table 2.25: Pathogens capable of crossing the placental barrier (TORCH infections)
Adhesion
Following the initial exposure, the pathogen adheres at the portal of entry. The term adhesion refers to the capability of pathogenic microbes to attach to the cells of the body using adhesion factors, and different pathogens use various mechanisms to adhere to the cells of host tissues.
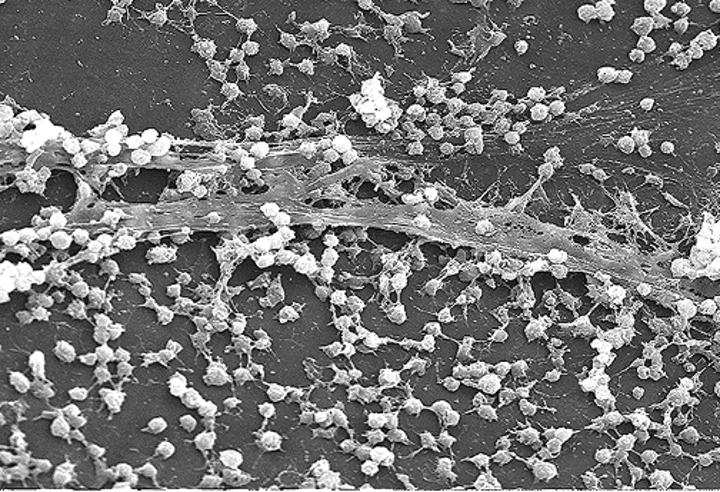
Molecules (either proteins or carbohydrates) called adhesins are found on the surface of certain pathogens and bind to specific receptors (glycoproteins) on host cells. Adhesins are present on the fimbriae and flagella of bacteria, the cilia of protozoa, and the capsids or membranes of viruses. Protozoans can also use hooks and barbs for adhesion; spike proteins on viruses also enhance viral adhesion. The production of glycocalyces (slime layers and capsules) (figure 2.89), with their high sugar and protein content, can also allow certain bacterial pathogens to attach to cells.
Biofilm growth can also act as an adhesion factor. A biofilm is a community of bacteria that produce a glycocalyx, known as extra polymeric substance (EPS), that allows the biofilm to attach to a surface. Persistent Pseudomonas aeruginosa infections are common in patients suffering from cystic fibrosis, burn wounds, and middle-ear infections (otitis media) because P. aeruginosa produces a biofilm. The EPS allows the bacteria to adhere to the host cells and makes it harder for the host to physically remove the pathogen. The EPS not only allows for attachment but provides protection against the immune system and antibiotic treatments, preventing antibiotics from reaching the bacterial cells within the biofilm. In addition, not all bacteria in a biofilm are rapidly growing; some are in a stationary phase. Since antibiotics are most effective against rapidly growing bacteria, portions of bacteria in a biofilm are protected against antibiotics.[23]
Invasion
Once adhesion is successful, invasion can proceed. Invasion involves the dissemination of a pathogen throughout local tissues or the body. Pathogens may produce exoenzymes or toxins, which serve as virulence factors that allow them to colonize and damage host tissues as they spread deeper into the body. Pathogens may also produce virulence factors that protect them against immune system defenses. A pathogen’s specific virulence factors determine the degree of tissue damage that occurs. Figure 2.90 shows the invasion of H. pylori into the tissues of the stomach, causing damage as it progresses.
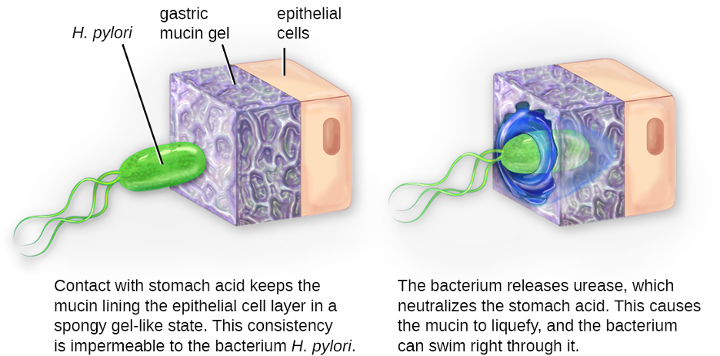
Intracellular pathogens achieve invasion by entering the host’s cells and reproducing. Some are obligate intracellular pathogens (meaning they can only reproduce inside of host cells) and others are facultative intracellular pathogens (meaning they can reproduce either inside or outside of host cells). By entering the host cells, intracellular pathogens are able to evade some mechanisms of the immune system while also exploiting the nutrients in the host cell.
Entry to a cell can occur by endocytosis. For most kinds of host cells, pathogens use one of two different mechanisms for endocytosis and entry. One mechanism relies on effector proteins secreted by the pathogen; these effector proteins trigger entry into the host cell. This is the method that Salmonella and Shigella use when invading intestinal epithelial cells. When these pathogens come in contact with epithelial cells in the intestine, they secrete effector molecules that cause protrusions of membrane ruffles that bring the bacterial cell in. This process is called membrane ruffling. The second mechanism relies on surface proteins expressed on the pathogen that bind to receptors on the host cell, resulting in entry. For example, Yersinia pseudotuberculosis produces a surface protein known as invasin that binds to beta-1 integrins expressed on the surface of host cells.
Some host cells, such as white blood cells and other phagocytes of the immune system, actively endocytose pathogens in a process called phagocytosis. Although phagocytosis allows the pathogen to gain entry to the host cell, in most cases, the host cell kills and degrades the pathogen by using digestive enzymes. Normally, when a pathogen is ingested by a phagocyte, it is enclosed within a phagosome in the cytoplasm; the phagosome fuses with a lysosome to form a phagolysosome, where digestive enzymes kill the pathogen (see section 1.6). However, some intracellular pathogens have the ability to survive and multiply within phagocytes. Examples include Listeria monocytogenes and Shigella; these bacteria produce proteins that lyse the phagosome before it fuses with the lysosome, allowing the bacteria to escape into the phagocyte’s cytoplasm where they can multiply. Bacteria such as Mycobacterium tuberculosis, Legionella pneumophila, and Salmonella species use a slightly different mechanism to evade being digested by the phagocyte. These bacteria prevent the fusion of the phagosome with the lysosome, thus remaining alive and dividing within the phagosome.
Infection
Following invasion, successful multiplication of the pathogen leads to infection. Infections can be described as local, focal, or systemic depending on the extent of the infection. A local infection is confined to a small area of the body, typically near the portal of entry. For example, a hair follicle infected by Staphylococcus aureus infection may result in a boil around the site of infection, but the bacterium is largely contained to this small location. Other examples of local infections that involve more extensive tissue involvement include urinary tract infections confined to the bladder or pneumonia confined to the lungs.
In a focal infection, a localized pathogen, or the toxins it produces, can spread to a secondary location. For example, a dental hygienist nicking the gum with a sharp tool can lead to a local infection in the gum by Streptococcus bacteria of the normal oral microbiota. These Streptococcus spp. may then gain access to the bloodstream and make their way to other locations in the body, resulting in a secondary infection.
When an infection becomes disseminated throughout the body, we call it a systemic infection. For example, infection by the varicella-zoster virus typically gains entry through a mucous membrane of the upper respiratory system. It then spreads throughout the body, resulting in the classic red skin lesions associated with chickenpox. Since these lesions are not sites of initial infection, they are signs of a systemic infection.
Sometimes a primary infection, the initial infection caused by one pathogen, can lead to a secondary infection by another pathogen. For example, the immune system of a patient with the primary infection HIV becomes compromised, making the patient more susceptible to secondary diseases like oral thrush and others caused by opportunistic pathogens. Similarly, a primary infection by the influenza virus damages and decreases the defense mechanisms of the lungs, making patients more susceptible to an infection from secondary pneumonia caused by a bacterial pathogen like Haemophilus influenzae or Streptococcus pneumoniae. Some secondary infections can even develop as a result of treatment for a primary infection. Antibiotic therapy targeting the primary pathogen can cause collateral damage to the normal microbiota, creating an opening for opportunistic pathogens.
Transmission of Disease
For a pathogen to persist, it must put itself in a position to be transmitted to a new host, leaving the infected host through a portal of exit (figure 2.91). As with portals of entry, many pathogens are adapted to use a particular portal of exit. Similar to portals of entry, the most common portals of exit include the skin and the respiratory, urogenital, and gastrointestinal tracts. Coughing and sneezing can expel pathogens from the respiratory tract. A single sneeze can send thousands of virus particles into the air. Secretions and excretions can transport pathogens out of other portals of exit. Feces, urine, semen, vaginal secretions, tears, sweat, and shed skin cells can all serve as vehicles for a pathogen to leave the body. Pathogens that rely on insect vectors for transmission exit the body in the blood extracted by a biting insect. Similarly, some pathogens exit the body in blood extracted by needles.
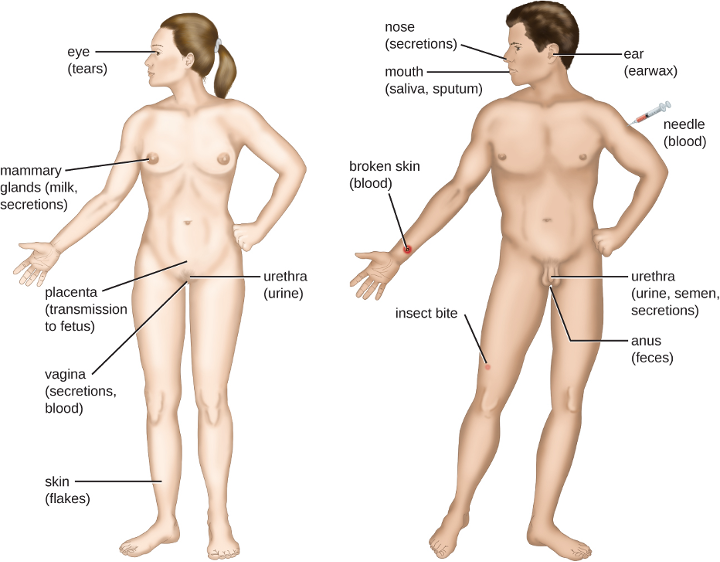
2.14 Virulence Factors of Bacterial and Viral Pathogens
Some pathogens are more virulent than others. This is due to the unique virulence factors produced by individual pathogens, which determine the extent and severity of disease they may cause. A pathogen’s virulence factors are encoded by genes that can be identified using molecular Koch’s postulates. When genes encoding virulence factors are inactivated, virulence in the pathogen is diminished. In this section, we examine various types and specific examples of virulence factors and how they contribute to each step of pathogenesis.
Virulence Factors for Adhesion
The first two steps in pathogenesis are exposure and adhesion. Recall that an adhesin is a protein or glycoprotein found on the surface of a pathogen that attaches to receptors on the host cell. Adhesins are found on bacterial, viral, fungal, and protozoan pathogens. One example of a bacterial adhesin is type 1 fimbrial adhesin, a molecule found on the tips of fimbriae of enterotoxigenic E. coli (ETEC). Recall that fimbriae are hairlike protein bristles on the cell surface. Type 1 fimbrial adhesin allows the fimbriae of ETEC cells to attach to the mannose glycans expressed on intestinal epithelial cells. Table 2.26 lists common adhesins found in some of the pathogens we have discussed or will be seeing later in this chapter.
Pathogen | Disease | Adhesin | Attachment Site |
---|---|---|---|
Streptococcus pyogenes | Strep throat | Protein F | Respiratory epithelial cells |
Streptococcus mutans | Dental caries | Adhesin P1 | Teeth |
Neisseria gonorrhoeae | Gonorrhea | Type IV pili | Urethral epithelial cells |
Enterotoxigenic E. coli (ETEC) | Traveler’s diarrhea | Type 1 fimbriae | Intestinal epithelial cells |
Vibrio cholerae | Cholera | N-methylphenylalanine pili | Intestinal epithelial cells |
Table 2.26: Some bacterial adhesins and their host attachment sites
Bacterial Exoenzymes and Toxins as Virulence Factors
After exposure and adhesion, the next step in pathogenesis is invasion, which can involve enzymes and toxins. Many pathogens achieve invasion by entering the bloodstream, an effective means of dissemination because blood vessels pass close to every cell in the body. The downside of this mechanism of dispersal is that the blood also includes numerous elements of the immune system. Various terms ending in –emia are used to describe the presence of pathogens in the bloodstream. The presence of bacteria in blood is called bacteremia. Bacteremia involving pyogenes (pus-forming bacteria) is called pyemia. When viruses are found in the blood, it is called viremia. The term toxemia describes the condition when toxins are found in the blood. If bacteria are both present and multiplying in the blood, this condition is called septicemia.
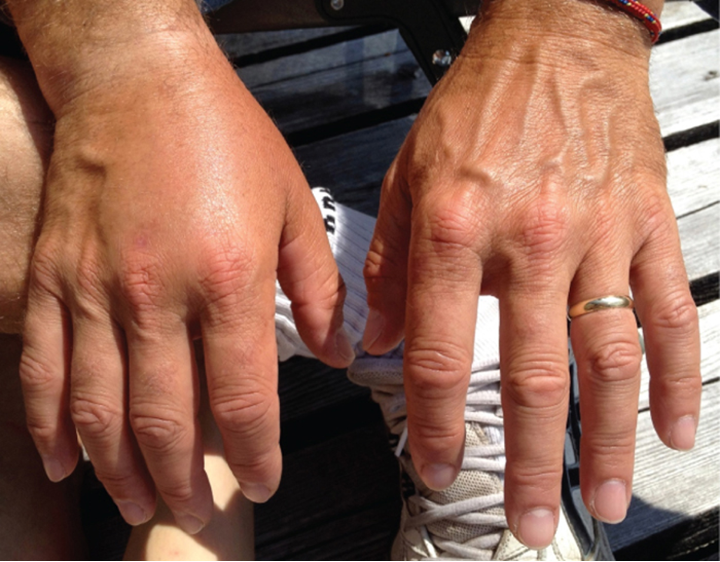
Patients with septicemia are described as septic, which can lead to shock, a life-threatening decrease in blood pressure (systolic pressure <90 mm Hg) that prevents cells and organs from receiving enough oxygen and nutrients. Some bacteria can cause shock through the release of toxins (virulence factors that can cause tissue damage) and lead to low blood pressure. Gram-negative bacteria are engulfed by immune system phagocytes, which then release tumor necrosis factor, a molecule involved in inflammation and fever. Tumor necrosis factor binds to blood capillaries to increase their permeability, allowing fluids to pass out of blood vessels and into tissues, causing swelling, or edema (figure 2.92). With high concentrations of tumor necrosis factor, the inflammatory reaction is severe and enough fluid is lost from the circulatory system that blood pressure decreases to dangerously low levels. This can have dire consequences because the heart, lungs, and kidneys rely on normal blood pressure for proper function; thus, multi-organ failure, shock, and death can occur.
Exoenzymes
Some pathogens produce extracellular enzymes, or exoenzymes, that enable them to invade host cells and deeper tissues. Exoenzymes have a wide variety of targets. Some general classes of exoenzymes and associated pathogens are listed in table 2.27. Each of these exoenzymes functions in the context of a particular tissue structure to facilitate invasion or support its own growth and defend against the immune system. For example, hyaluronidase S, an enzyme produced by pathogens like Staphylococcus aureus, Streptococcus pyogenes, and Clostridium perfringens, degrades the glycoside hyaluronan (hyaluronic acid), which acts as an intercellular cement between adjacent cells in connective tissue (figure 2.93). This allows the pathogen to pass through the tissue layers at the portal of entry and disseminate elsewhere in the body (figure 2.93).
Class | Example | Function |
---|---|---|
Glycohydrolases | Hyaluronidase S in Staphylococcus aureus | Degrades hyaluronic acid that cements cells together to promote spreading through tissues |
Nucleases | DNAse produced by S. aureus | Degrades DNA released by dying cells (bacteria and host cells) that can trap the bacteria, thus promoting spread |
Phospholipases | Phospholipase C of Bacillus anthracis | Degrades phospholipid bilayer of host cells, causing cellular lysis, and degrade membrane of phagosomes to enable escape into the cytoplasm |
Proteases | Collagenase in Clostridium perfringens | Degrades collagen in connective tissue to promote spread |
Table 2.27: Some classes of exoenzymes and their targets
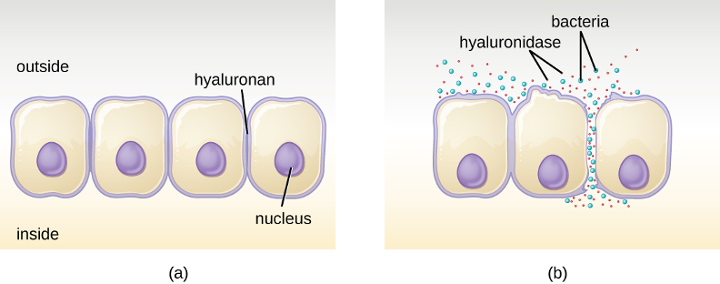
Pathogen-produced nucleases, such as DNAse produced by S. aureus, degrade extracellular DNA as a means of escape and spreading through tissue. As bacterial and host cells die at the site of infection, they lyse and release their intracellular contents. The DNA chromosome is the largest of the intracellular molecules, and masses of extracellular DNA can trap bacteria and prevent their spread. S. aureus produces a DNAse to degrade the mesh of extracellular DNA so it can escape and spread to adjacent tissues. This strategy is also used by S. aureus and other pathogens to degrade and escape webs of extracellular DNA produced by immune system phagocytes to trap the bacteria.
Enzymes that degrade the phospholipids of cell membranes are called phospholipases. Their actions are specific in regard to the type of phospholipids they act upon and where they enzymatically cleave the molecules. The pathogen responsible for anthrax, B. anthracis, produces phospholipase C. When B. anthracis is ingested by phagocytic cells of the immune system, phospholipase C degrades the membrane of the phagosome before it can fuse with the lysosome, allowing the pathogen to escape into the cytoplasm and multiply. Phospholipases can also target the membrane that encloses the phagosome within phagocytic cells. As described earlier in this chapter, this is the mechanism used by intracellular pathogens such as L. monocytogenes and Rickettsia to escape the phagosome and multiply within the cytoplasm of phagocytic cells. The role of phospholipases in bacterial virulence is not restricted to phagosomal escape. Many pathogens produce phospholipases that act to degrade cell membranes and cause lysis of target cells. These phospholipases are involved in lysis of red blood cells, white blood cells, and tissue cells.
Bacterial pathogens also produce various protein-digesting enzymes, or proteases. Proteases can be classified according to their substrate target (e.g., serine proteases target proteins with the amino acid serine) or if they contain metals in their active site (e.g., zinc metalloproteases contain a zinc ion, which is necessary for enzymatic activity).
Toxins
In addition to exoenzymes, certain pathogens are able to produce toxins, biological poisons that assist in their ability to invade and cause damage to tissues. The ability of a pathogen to produce toxins to cause damage to host cells is called toxigenicity.
Toxins can be categorized as endotoxins or exotoxins. The lipopolysaccharide (LPS) found on the outer membrane of gram-negative bacteria is called endotoxin (figure 2.94). During infection and disease, gram-negative bacterial pathogens release endotoxin either when the cell dies, resulting in the disintegration of the membrane, or when the bacterium undergoes binary fission. The lipid component of endotoxin, lipid A, is responsible for the toxic properties of the LPS molecule. Lipid A is relatively conserved across different genera of gram-negative bacteria; therefore, the toxic properties of lipid A are similar regardless of the gram-negative pathogen. In a manner similar to that of tumor necrosis factor, lipid A triggers the immune system’s inflammatory response (see section 1.7). If the concentration of endotoxin in the body is low, the inflammatory response may provide the host an effective defense against infection; on the other hand, high concentrations of endotoxin in the blood can cause an excessive inflammatory response, leading to a severe drop in blood pressure, multi-organ failure, and death.

A classic method of detecting endotoxin is by using the Limulus amebocyte lysate (LAL) test. In this procedure, the blood cells (amebocytes) of the horseshoe crab (Limulus polyphemus) are mixed with a patient’s serum. The amebocytes will react to the presence of any endotoxin. This reaction can be observed either chromogenically (color) or by looking for coagulation (clotting reaction) to occur within the serum. An alternative method that has been used is an enzyme-linked immunosorbent assay (ELISA) that uses antibodies to detect the presence of endotoxin.
Unlike the toxic lipid A of endotoxin, exotoxins are protein molecules that are produced by a wide variety of living pathogenic bacteria. Although some gram-negative pathogens produce exotoxins, the majority are produced by gram-positive pathogens. Exotoxins differ from endotoxin in several other key characteristics, summarized in table 2.28. In contrast to endotoxin, which stimulates a general systemic inflammatory response when released, exotoxins are much more specific in their action and the cells they interact with. Each exotoxin targets specific receptors on specific cells and damages those cells through unique molecular mechanisms. Endotoxin remains stable at high temperatures, and requires heating at 121 °C (250 °F) for 45 minutes to inactivate. By contrast, most exotoxins are heat labile because of their protein structure, and many are denatured (inactivated) at temperatures above 41 °C (106 °F). As discussed earlier, endotoxin can stimulate a lethal inflammatory response at very high concentrations and has a measured LD50 of 0.24 mg/kg. By contrast, very small concentrations of exotoxins can be lethal. For example, botulinum toxin, which causes botulism, has an LD50 of 0.000001 mg/kg (240,000 times more lethal than endotoxin).
Characteristic | Endotoxin | Exotoxin |
---|---|---|
Source | Gram-negative bacteria | Gram-positive (primarily) and gram-negative bacteria |
Composition | Lipid A component of lipopolysaccharide | Protein |
Effect on host | General systemic symptoms of inflammation and fever | Specific damage to cells dependent upon receptor-mediated targeting of cells and specific mechanisms of action |
Heat stability | Heat stable | Most are heat labile, but some are heat stable |
LD50 | High | Low |
Table 2.28: Comparison of endotoxin and exotoxins produced by bacteria
The exotoxins can be grouped into three categories based on their target: intracellular targeting, membrane disrupting, and superantigens. Table 2.29 provides examples of well-characterized toxins within each of these three categories.
The intracellular targeting toxins comprise two components: A for activity and B for binding. Thus, these types of toxins are known as A-B exotoxins (figure 2.95). The B component is responsible for the cellular specificity of the toxin and mediates the initial attachment of the toxin to specific cell surface receptors. Once the A-B toxin binds to the host cell, it is brought into the cell by endocytosis and entrapped in a vacuole. The A and B subunits separate as the vacuole acidifies. The A subunit then enters the cell cytoplasm and interferes with the specific internal cellular function that it targets.
Category | Example | Pathogen | Mechanism and Disease |
---|---|---|---|
Intracellular-targeting toxins | Cholera toxin | Vibrio cholerae | Activation of adenylate cyclase in intestinal cells, causing increased levels of cyclic adenosine monophosphate (cAMP) and secretion of fluids and electrolytes out of cell, causing diarrhea |
Tetanus toxin | Clostridium tetani | Inhibits the release of inhibitory neurotransmitters in the central nervous system, causing spastic paralysis | |
Botulinum toxin | Clostridium botulinum | Inhibits release of the neurotransmitter acetylcholine from neurons, resulting in flaccid paralysis | |
Diphtheria toxin | Corynebacterium diphtheriae | Inhibition of protein synthesis, causing cellular death | |
Membrane-disrupting toxins | Streptolysin | Streptococcus pyogenes | Proteins that assemble into pores in cell membranes, disrupting their function and killing the cell |
Pneumolysin | Streptococcus pneumoniae | ||
Alpha-toxin | Staphylococcus aureus | ||
Alpha-toxin | Clostridium perfringens | Phospholipases that degrade cell membrane phospholipids, disrupting membrane function and killing the cell | |
Phospholipase C | Pseudomonas aeruginosa | ||
Beta-toxin | Staphylococcus aureus | ||
Superantigens | Toxic shock syndrome toxin | Staphylococcus aureus | Stimulates excessive activation of immune system cells and release of cytokines (chemical mediators) from immune system cells. Life-threatening fever, inflammation, and shock are the result. |
Streptococcal mitogenic exotoxin | Streptococcus pyogenes | ||
Streptococcal pyrogenic toxins | Streptococcus pyogenes |
Table 2.29: Some common exotoxins and associated bacterial pathogens
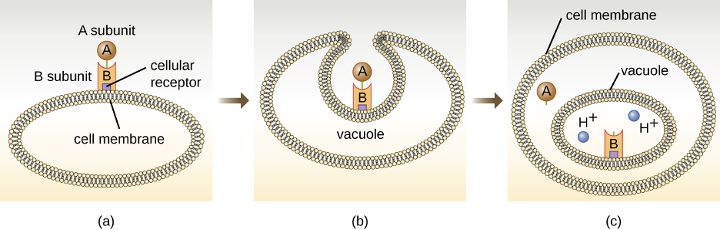
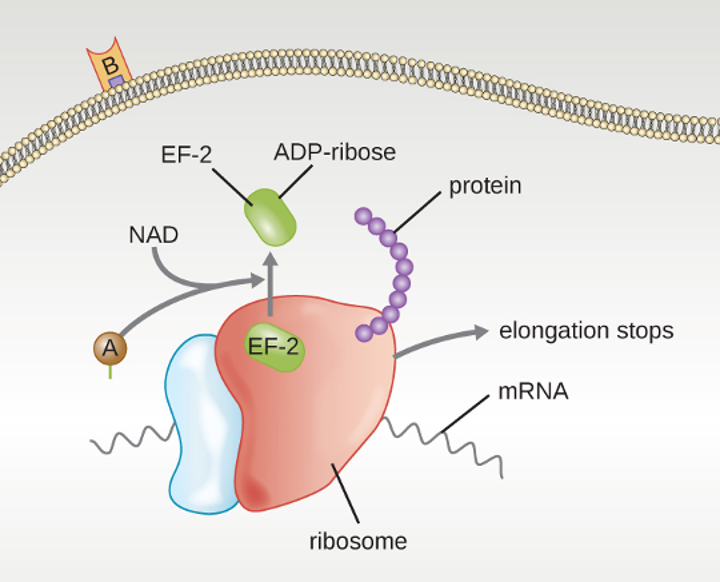
Four unique examples of A-B toxins are the diphtheria, cholera, botulinum, and tetanus toxins. The diphtheria toxin is produced by the gram-positive bacterium Corynebacterium diphtheriae, the causative agent of nasopharyngeal and cutaneous diphtheria. After the A subunit of the diphtheria toxin separates and gains access to the cytoplasm, it facilitates the transfer of adenosine diphosphate (ADP)-ribose onto an elongation-factor protein (EF-2) that is needed for protein synthesis. Hence, diphtheria toxin inhibits protein synthesis in the host cell, ultimately killing the cell (figure 2.96).
Cholera toxin is an enterotoxin produced by the gram-negative bacterium Vibrio cholerae and is composed of one A subunit and five B subunits. The mechanism of action of the cholera toxin is complex. The B subunits bind to receptors on the intestinal epithelial cell of the small intestine. After gaining entry into the cytoplasm of the epithelial cell, the A subunit activates an intracellular G protein. The activated G protein, in turn, leads to the activation of the enzyme adenylyl cyclase, which begins to produce an increase in the concentration of cyclic AMP (a secondary messenger molecule). The increased cAMP disrupts the normal physiology of the intestinal epithelial cells and causes them to secrete excessive amounts of fluid and electrolytes into the lumen of the intestinal tract, resulting in severe “rice-water stool” diarrhea characteristic of cholera.
Botulinum toxin (also known as botox) is a neurotoxin produced by the gram-positive bacterium Clostridium botulinum. It is the most acutely toxic substance known to date. The toxin is composed of a light A subunit and heavy protein chain B subunit. The B subunit binds to neurons to allow botulinum toxin to enter the neurons at the neuromuscular junction. The A subunit acts as a protease, cleaving proteins involved in the neuron’s release of acetylcholine, a neurotransmitter molecule. Normally, neurons release acetylcholine to induce muscle fiber contractions. The toxin’s ability to block acetylcholine release results in the inhibition of muscle contractions, leading to muscle relaxation. This has the potential to stop breathing and cause death. Because of its action, low concentrations of botox are used for cosmetic and medical procedures, including the removal of wrinkles and treatment of overactive bladder.
Another neurotoxin is tetanus toxin, which is produced by the gram-positive bacterium Clostridium tetani. This toxin also has a light A subunit and heavy protein chain B subunit. Unlike botulinum toxin, tetanus toxin binds to inhibitory interneurons, which are responsible for release of the inhibitory neurotransmitters glycine and gamma-aminobutyric acid (GABA). Normally, these neurotransmitters bind to neurons at the neuromuscular junction, resulting in the inhibition of acetylcholine release. Tetanus toxin inhibits the release of glycine and GABA from the interneuron, resulting in permanent muscle contraction. The first symptom is typically stiffness of the jaw (lockjaw). Violent muscle spasms in other parts of the body follow, typically culminating with respiratory failure and death. Figure 2.97 shows the actions of both botulinum and tetanus toxins.
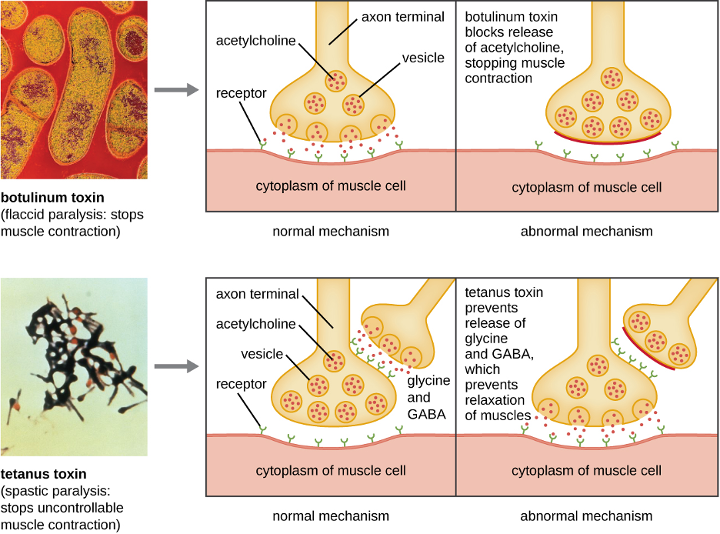
Membrane-disrupting toxins affect cell membrane function either by forming pores or by disrupting the phospholipid bilayer in host cell membranes. Two types of membrane-disrupting exotoxins are hemolysins and leukocidins, which form pores in cell membranes, causing leakage of the cytoplasmic contents and cell lysis. These toxins were originally thought to target red blood cells (erythrocytes) and white blood cells (leukocytes), respectively, but we now know they can affect other cells as well. The gram-positive bacterium Streptococcus pyogenes produces streptolysins, water-soluble hemolysins that bind to the cholesterol moieties in the host cell membrane to form a pore. The two types of streptolysins, O and S, are categorized by their ability to cause hemolysis in erythrocytes in the absence or presence of oxygen. Streptolysin O is not active in the presence of oxygen, whereas streptolysin S is active in the presence of oxygen. Other important pore-forming membrane-disrupting toxins include alpha toxin of Staphylococcus aureus and pneumolysin of Streptococcus pneumoniae.
Bacterial phospholipases are membrane-disrupting toxins that degrade the phospholipid bilayer of cell membranes rather than forming pores. We have already discussed the phospholipases associated with B. anthracis, L. pneumophila, and Rickettsia species that enable these bacteria to affect the lysis of phagosomes. These same phospholipases are also hemolysins. Other phospholipases that function as hemolysins include the alpha toxin of Clostridium perfringens, phospholipase C of P. aeruginosa, and beta toxin of Staphylococcus aureus.
Some strains of S. aureus also produce a leukocidin called Panton-Valentine leukocidin (PVL). PVL consists of two subunits, S and F. The S component acts like the B subunit of an A-B exotoxin in that it binds to glycolipids on the outer plasma membrane of animal cells. The F-component acts like the A subunit of an A-B exotoxin and carries the enzymatic activity. The toxin inserts and assembles into a pore in the membrane. Genes that encode PVL are more frequently present in S. aureus strains that cause skin infections and pneumonia.[24] PVL promotes skin infections by causing edema, erythema (reddening of the skin due to blood vessel dilation), and skin necrosis. PVL has also been shown to cause necrotizing pneumonia. PVL promotes pro-inflammatory and cytotoxic effects on alveolar leukocytes. This results in the release of enzymes from the leukocytes, which, in turn, cause damage to lung tissue.
The third class of exotoxins is the superantigens. These are exotoxins that trigger an excessive, nonspecific stimulation of immune cells to secrete cytokines (chemical messengers). The excessive production of cytokines, often called a cytokine storm, elicits a strong immune and inflammatory response that can cause life-threatening high fevers, low blood pressure, multi-organ failure, shock, and death. The prototype superantigen is the toxic shock syndrome toxin of S. aureus. Most toxic shock syndrome cases are associated with vaginal colonization by toxin-producing S. aureus in menstruating women; however, colonization of other body sites can also occur. Some strains of Streptococcus pyogenes also produce superantigens; they are referred to as the streptococcal mitogenic exotoxins and the streptococcal pyrogenic toxins.
Virulence Factors for Survival in the Host and Immune Evasion
Evading the immune system is also important to invasiveness. Bacteria use a variety of virulence factors to evade phagocytosis by cells of the immune system. For example, many bacteria produce capsules, which are used in adhesion but also aid in immune evasion by preventing ingestion by phagocytes. The composition of the capsule prevents immune cells from being able to adhere and then phagocytose the cell. In addition, the capsule makes the bacterial cell much larger, making it harder for immune cells to engulf the pathogen (figure 2.100). A notable capsule-producing bacterium is the gram-positive pathogen Streptococcus pneumoniae, which causes pneumococcal pneumonia, meningitis, septicemia, and other respiratory tract infections. Encapsulated strains of S. pneumoniae are more virulent than nonencapsulated strains and are more likely to invade the bloodstream and cause septicemia and meningitis.
Some pathogens can also produce proteases to protect themselves against phagocytosis. The human immune system produces antibodies that bind to surface molecules found on specific bacteria (e.g., capsules, fimbriae, flagella, LPS). This binding initiates phagocytosis and other mechanisms of antibacterial killing and clearance. Proteases combat antibody-mediated killing and clearance by attacking and digesting the antibody molecules (figure 2.98).
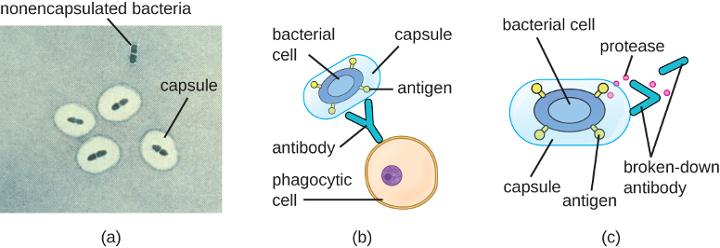
In addition to capsules and proteases, some bacterial pathogens produce other virulence factors that allow them to evade the immune system. The fimbriae of certain species of Streptococcus contain M protein, which alters the surface of Streptococcus and inhibits phagocytosis by blocking the binding of the complement molecules that assist phagocytes in ingesting bacterial pathogens. The acid-fast bacterium Mycobacterium tuberculosis (the causative agent of tuberculosis) produces a waxy substance known as mycolic acid in its cell envelope. When it is engulfed by phagocytes in the lung, the protective mycolic acid coat enables the bacterium to resist some of the killing mechanisms within the phagolysosome.
Some bacteria produce virulence factors that promote infection by exploiting molecules naturally produced by the host. For example, most strains of Staphylococcus aureus produce the exoenzyme coagulase, which exploits the natural mechanism of blood clotting to evade the immune system. Normally, blood clotting is triggered in response to blood vessel damage; platelets begin to plug the clot, and a cascade of reactions occurs in which fibrinogen, a soluble protein made by the liver, is cleaved into fibrin. Fibrin is an insoluble, thread-like protein that binds to blood platelets, cross-links, and contracts to form a mesh of clumped platelets and red blood cells. The resulting clot prevents further loss of blood from the damaged blood vessels. However, if bacteria release coagulase into the bloodstream, the fibrinogen-to-fibrin cascade is triggered in the absence of blood vessel damage. The resulting clot coats the bacteria in fibrin, protecting the bacteria from exposure to phagocytic immune cells circulating in the bloodstream.
Whereas coagulase causes blood to clot, kinases have the opposite effect by triggering the conversion of plasminogen to plasmin, which is involved in the digestion of fibrin clots. By digesting a clot, kinases allow pathogens trapped in the clot to escape and spread, similar to the way that collagenase, hyaluronidase, and DNAse facilitate the spread of infection. Examples of kinases include staphylokinases and streptokinases, produced by Staphylococcus aureus and Streptococcus pyogenes, respectively. It is intriguing that S. aureus can produce both coagulase to promote clotting and staphylokinase to stimulate the digestion of clots. The action of the coagulase provides an important protective barrier from the immune system, but when nutrient supplies are diminished or other conditions signal a need for the pathogen to escape and spread, the production of staphylokinase can initiate this process.
A final mechanism that pathogens can use to protect themselves against the immune system is called antigenic variation, which is the alteration of surface proteins so that a pathogen is no longer recognized by the host’s immune system. For example, the bacterium Borrelia burgdorferi, the causative agent of Lyme disease, contains a surface lipoprotein known as VlsE. Because of genetic recombination during DNA replication and repair, this bacterial protein undergoes antigenic variation. Each time fever occurs, the VlsE protein in B. burgdorferi can differ so much that antibodies against previous VlsE sequences are not effective. It is believed that this variation in the VlsE contributes to the ability of B. burgdorferi to cause chronic disease. Another important human bacterial pathogen that uses antigenic variation to avoid the immune system is Neisseria gonorrhoeae, which causes the sexually transmitted disease gonorrhea. This bacterium is well known for its ability to undergo antigenic variation of its type IV pili to avoid immune defenses.
Viral Virulence
Although viral pathogens are not similar to bacterial pathogens in terms of structure, some of the properties that contribute to their virulence are similar. Viruses use adhesins to facilitate adhesion to host cells, and certain enveloped viruses rely on antigenic variation to avoid the host immune defenses. These virulence factors are discussed in more detail in the following sections.
Viral Adhesins
One of the first steps in any viral infection is adhesion of the virus to specific receptors on the surface of cells. This process is mediated by adhesins that are part of the viral capsid or membrane envelope. The interaction of viral adhesins with specific cell receptors defines the tropism (preferential targeting) of viruses for specific cells, tissues, and organs in the body. The spike protein hemagglutinin found on the influenza virus is an example of a viral adhesin; it allows the virus to bind to the sialic acid on the membrane of host respiratory and intestinal cells. Another viral adhesin is the glycoprotein gp20, found on HIV. For HIV to infect cells of the immune system, it must interact with two receptors on the surface of cells. The first interaction involves binding between gp120 and the CD4 cellular marker that is found on some essential immune system cells. However, before viral entry into the cell can occur, a second interaction between gp120 and one of two chemokine receptors (CCR5 and CXCR4) must occur. Table 2.30 lists the adhesins for some common viral pathogens and the specific sites to which these adhesins allow viruses to attach.
Pathogen | Disease | Adhesin | Attachment Site |
---|---|---|---|
Influenza virus | Influenza | Hemagglutinin | Sialic acid of respiratory and intestinal cells |
Herpes simplex virus I or II | Oral herpes, genital herpes | Glycoproteins gB, gC, gD | Heparan sulfate on mucosal surfaces of the mouth and genitals |
Human immunodeficiency virus | HIV/AIDS | Glycoprotein gp120 | CD4 and CCR5 or CXCR4 of immune system cells |
Table 2.30: Some viral adhesins and their host attachment sites
Antigenic Variation in Viruses
Antigenic variation also occurs in certain types of enveloped viruses, including influenza viruses, which exhibit two forms of antigenic variation: antigenic drift and antigenic shift (figure 2.99). Antigenic drift is the result of point mutations causing slight changes in the spike proteins hemagglutinin (H) and neuraminidase (N). On the other hand, antigenic shift is a major change in spike proteins due to gene reassortment. This reassortment for antigenic shift occurs typically when two different influenza viruses infect the same host.
The rate of antigenic variation in influenza viruses is very high, making it difficult for the immune system to recognize the many different strains of influenza virus. Although the body may develop immunity to one strain through natural exposure or vaccination, antigenic variation results in the continual emergence of new strains that the immune system will not recognize. This is the main reason that vaccines against the influenza virus must be given annually. Each year’s influenza vaccine provides protection against the most prevalent strains for that year, but new or different strains may be more prevalent the following year.
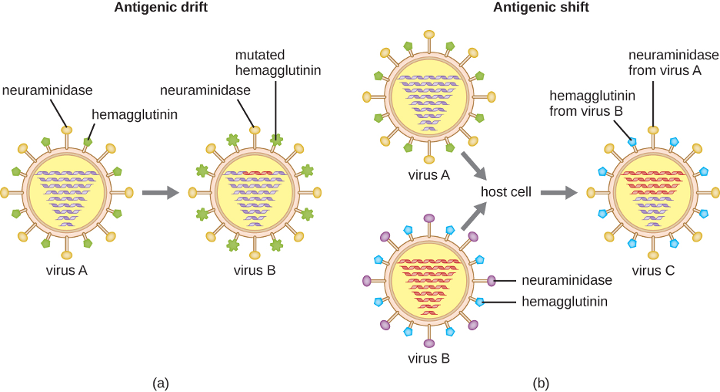
Figure Descriptions
Figure 2.1: A diagram of a rod-shaped prokaryotic cell. The thick outer layer is called the capsule, inside of that is a thinner cell wall and inside of that is an even thinner plasma membrane. Inside of the plasma membrane is a fluid called the cytoplasm, little dots called ribosomes, small spheres called inclusions, a small loop of DNA called a plasmid, and a large folded loop of DNA called the nucleoid. Long projections start at the plasma membrane and extend out of the capsule; these are called flagella (singular: flagellum). A shorter projection is labeled pilus. And many very short projections are labeled fimbriae.
Figure 2.2: A micrograph of an oval cell with a lighter region in the center of the cell. The lighter region takes up approximately one third of the volume of the cell and is labeled nucleoid.
Figure 2.3: There are three prokaryote-specific mechanisms leading to horizontal gene transfer in prokaryotes. a) In transformation, the cell takes up DNA directly from the environment. The DNA may remain separate as a plasmid or be incorporated into the host genome. b) In transduction, a bacteriophage injects DNA that is a hybrid of viral DNA and DNA from a previously infected bacterial cell. c) In conjugation, DNA is transferred between cells through a cytoplasmic bridge after a conjugation pilus draws the two cells close enough to form the bridge.
Figure 2.4: A drawing showing that the complete ribosome is made of a small subunit and a large subunit. The small subunit is about half the size of the large one. The small subunit has a size of 30S, the large subunit has a size of 50S and the complete ribosome (containing both the small and large subunit) has a size of 70S.
Figure 2.5: a) A micrograph showing gray spheres each containing 2-8 smaller white spheres. The gray spheres are approximately 600 nm in diameter B) A micrograph showing thin ribbons of approximately 100 µm length; each ribbon contains many dark spots in a line down the center of the ribbon. C) A micrograph showing a gray sphere of approximately 4 µm diameter with a cluster of smaller white spheres at the bottom of the larger sphere. D) A micrograph showing a larger sphere of approximately 10 µm diameter with many smaller spheres of approximately 1 µm diameter inside of the larger sphere. 3) a micrograph showing a long ribbon over 500 nm in length with small dots in the center. A closeup shows the dots to be a chain of spheres approximately 20 nm in diameter.
Figure 2.6: a) A diagram showing the process of sporulation. Step 1 – the DNA replicates. The image shows a rod shaped cell with 2 loops of DNA; one in the center and one towards the end of the cell. Step 2 – Membranes form around the DNA. The drawing shows lines encircling the loop of DNA at the end of the cell. Step 3 – Forespore forms additional membranes. The lines around the loop of DNA are thickened. Step 4 – Protective cortex forms around the spore. The lines around the loop of DNA are thickened even more. Step 5 – protein coat forma around the cortex. The lines around the loop of DNA are thickened even more and the outer cell lyses. Step 6 – the endospore is released. A small spherical structure with DNA inside of many thick layers is shown. B) A micrograph of an endospore shows a dark central core inside a lighter region; these are surrounded by thick layers on the outside. C) a micrograph showing red rods in chains; many of the rods have a green dot in their center.
Figure 2.7: The diagram of the gram-positive cell wall shows alternative NAG (N-acetylglucosamine) and NAM (N-acetylmuramic acid) in a chain; these are shown as alternative red and blue spheres. The chains or red and blue spheres are connected to other chains with smaller yellow spheres in a chain labeled pentapeptide and smaller green spheres labeled tetrapeptide. Each NAG in the chain is connected to the NAG in the chains next to it by both a tetrapeptide connected to a pentapeptide. The diagram of the gram-negative cell wall has the same NAG and NAM chains. But this time, they are linked with a direct line to the chains next to them.
Figure 2.8: The gram-positive bacterial cell wall diagram shows a plasma membrane on top of the cytoplasm. The cell wall is shown as a thick layer of peptidoglycans connected to the plasma membrane by teichoic acids. The gram-negative cell wall also has a plasma membrane on top of the cytoplasm. On top of the plasma membrane is a thin periplasmic space. On top of that is a thin peptidoglycan cell wall. On top of that is an outer membrane that contains murein lipoproteins that connect the outer membrane to the peptidoglycan cell wall. Lipid A, O antigens, and lipopolysaccharides sit on top of the outer membrane. Proins are tubes that connect the outside of the outer membrane with the region of the peptidoglycan cell wall.
Figure 2.9: A) A diagram of gram-positive acid-fast bacteria. The plasma membrane is shown on top of the cytoplasm and a thick layer of peptidoglycan makes up the cell wall outside the plasma membrane. Teichoic acids connect the peptidoglycans to the plasma membrane. On top of the peptidoglycans are mycolic acids, lipomannan, and arabinoglycans. B) A micrograph of red cells labeled acid-fast bacteria.
Figure 2.10: A diagram of the outer membrane of gram-negative bacteria. At the top of the diagram is a long chain of structures labeled O antigen. Below that is a shorter chain labeled core. Below that are two spheres labeled lipid A. Attached to the lipid A are squiggly tails labeled fatty acids.
Figure 2.11: a) A diagram showing the outer structures of bacterial cells. The thick outer layer is labeled capsule. Below that is a thinner cell wall and below that is an even thinner plasma membrane. B) A micrograph showing capsules as clearings outside of red stained cells; the background of the micrograph is a pale pink.
Figure 2.12: a) A diagram showing the outer structures of bacterial cells. The thick outer layer is labeled capsule. Below that is a thinner cell wall and below that is an even thinner plasma membrane. B) A micrograph showing capsules as clearings outside of red stained cells; the background of the micrograph is a pale pink.
Figure 2.13: A diagram showing the attachment point of flagella in gram-positive and gram-negative bacteria. The gram-positive diagram shows the filament on the outside of the cell wall; a bent elbow labeled hook connects the filament to the cell wall. A thin line between the filament and hook is labeled junction. The hook connects to a rod which connects to a basal body in the inner membrane. The basal body is a complex structure with a C-ring on the bottom. In the center of this ring is a sphere labeled type III secretion protein. Outside of this are oval structures labeled stator. On top of the secretion protein is a ring labeled MS-ring. The gram-negative flagellum is similar. There is a filament attached to a junction attached to hook. In the outer membrane is a ring labeled L-ring that connects to a rod in the periplasmic space. A P-ring sits in the cell wall. In the inner membrane is the C-ring, type III secretion system, MS ring and stator.
Figure 2.14: Diagrams of flagellar arrangements. Monotrichous bacteria have a single flagellum at one end. Amphitrichouls bacteria have one flagellum at each end. Lophotrichous bacteria have a tuft of flagella at one end. Peritrichous bacteria have flagella all the way around the outside of the cell.
Figure 2.15: A diagram showing the run and tumble of bacterial motion. The tumble is when a clockwise rotation of flagella cause the bacterial cell to tumble about. The run is when a counter-clockwise rotation of the flagella cause the bacterial cell to move in a linear direction.
Figure 2.16: A micrograph shows purple circles and pink rods.
Figure 2.17: A micrograph shows red chains of cells on a blue background.
Figure 2.18: Micrograph a shows clear circles on a black background. Micrograph b shows red rods with a clear halo on a dark background.
Figure 2.19: A micrograph shows chains of red rods. Each red rod contains a green oval. An arrow pointing to the green ovals states: green endospore inside bacterial cells.
Figure 2.20: Three red rectangles on a clear background are shown. Each rectangle has many thin, wiggly lines projecting from it.
Figure 2.21: A) A micrograph of two rod shaped cells attached at their ends. B) A diagram of binary fission. First a cell replicates its DNA and elongates. Then, as the cell continues to elongate, each loop of DNA travels to one end or the other. The cell then starts to constrict in the center. This results in two cells each containing a loop of DNA.
Figure 2.22: A graph with time on the X axis and logarithm of living bacterial cells on the Y axis. The line of the graph begins towards the bottom of the Y axis and is flat for a short time. This is labeled 1) lag phase: no increase in number of living bacterial cells. Next the line slopes upwards. This is labeled Log phase: exponential increase in number of living bacterial cells. Next the line flattens again. This is labeled 3) Stationary phase: plateau in number of living bacterial cells; rate of cell division and death roughly equal. Final the line slopes downwards. This is labeled 4) Death or decline phase: exponential decrease in number of living bacterial cells.
Figure 2.23: a) An arithmetic scale graph showing log phase growth. The X axis is time (hours), and the Y axis is number of cells. The line on this graph starts fairly flat with readings of (1,2) and (5,32) but quickly slopes up steeply with a final reading of (10, 1024). B) is a semilog scale graph of log phase growth. The X axis is time (hours) and the Y axis is log 10 number of cells. The Y axis more clearly shows the difference between 10 superscript 1 and 10 superscript 3. The line on this graph is a straight line diagonally across the graph with points of (1,2), (5,32) ,and (10,1024) – just like the first graph. The equation for both graphs is N=2 superscript n.
Figure 2.24: A micrograph of blue cells labeled tick hemolymph cells. Inside of these cells are small red cells labeled R. rickettsia.
Figure 2.25: A photograph showing round domes on a brown background.
Figure 2.26: a) A photograph of shining green dots on a black background. B) A photograph of a glowing squid.
Figure 2.27: A) A micrograph of rod shaped cells. B) A photograph of an air conditioner.
Figure 2.28: A micrograph of many rod shaped cells.
Figure 2.29: An image of a round structure labeled fruiting body. Smaller spheres on this structure are labeled sporangium containing myxospores.
Figure 2.30: A micrograph of a rod shaped cell with many long projections.
Figure 2.31: A diagram showing the life cycle of Chlamydia. An epithelial cell is infected by small spheres labeled elementary bodies. Within 12 hours, these form into reticulate bodies which divide to form inclusions within 24 hours. Within the inclusions more elementary bodies are formed and within 72 hours these are released when the cell ruptures.
Figure 2.32: A light micrograph of long spiral shaped cells. A TEM cross-section of these shows a circle outlined by a cell membrane. Inside the cell is the cytoplasm and a darker region labeled nucleoid. Outside of this is the periplasmic space and outside of that is an outer membrane. A bulge within the periplasmic space is labeled axial filament. Small dots within the axial filament are labeled endoflagella. An SEM from the original light micrograph shows what looks like a thin rope wound around a thicker rope. The thin rope is labeled axial filament.
Figure 2.33: A micrograph of many rod shaped cells.
Figure 2.34: a) A micrograph of an oval cell with long projections attached to a root-shaped structure labeled holdfast. The oval cell is approximately 500 nm in diameter. B) A micrograph of a similar looking cell with a long projection that is not attached to a holdfast.
Figure 2.35: A thick glass tube filled with purple regions labeled purple bacteria and green regions labeled green bacteria.
Figure 2.36: a) A micrograph of green spherical cells. B) A photo of a green lake.
Figure 2.37: a) A micrograph of branched cells. B) A micrograph of cells arranged in a V-shape – these are labeled palisades. C) A micrograph of corn-flake shaped cells with a nucleus. Smaller cells outside of these are identified with an arrow.
Figure 2.38: A micrograph of many rod shaped cells.
Figure 2.39: a) A micrograph of spherical cells in a chain. B) A photograph of colonies on agar. The agar is red, and there is a clearing around each colony.
Figure 2.40: a) A micrograph of rod shaped cells in a chain. B) A photograph of colonies on agar. The agar is red and the colonies are white and fluffy looking.
Figure 2.41: A micrograph of clusters of spherical cells.
Figure 2.42: A photograph of colonies on agar. The agar is blue and the colonies look like a pile of beads.
Figure 2.43: Two photos of a rash on skin, the first of a large raised grayish ring, and the second of a raised red ring.
Figure 2.44: a) A micrograph of kite-shaped parasites. B) A single triangular parasite with multiple flagella.
Figure 2.45: Eimera life cycle. Environment sporogony is the process of sporulation occurring outside the host; this requires several days and oxygen. A non-infectious unsporulated oocyst becomes an infectious sporulated oocyst. These enter the gut when swallowed and begin the process of asexual schizogony. Oocysts release sporocysts which release sporozoites. Sporozoites invade gut cells and form trophozoites. Trophozoites undergo schizogony (asexual reproduction) to form schizont which releases merozoites. Merozoites can reinfect and become trophozoites again or continue with sexual gametogony where the merozoites form male and female gametes. The gametes undergo syngamy (sexual reproduction) to form a developing oocyst, which matures into an unsporulated non-infectious oocyst. This brings us back to the beginning of the environment sporogony stage of the cycle.
Figure 2.46: a) Paramecium cell with short strands on the outside labeled cilia. An indent in the outer layer is labeled cytostome. A sphere inside the cell at the base of the cytostome is labeled cytoproct. A star-shaped structure inside the cell is labeled contractile vacuole. B) Amoeba cell with projections on the outside labeled pseudopods. The outer layer of the cell is labeled ectoplasm and the inner layer is labeled endoplasm. A sphere inside the cell is labeled contractile vacuole. C) Euglena with a single long flagellum on the outside. The outer layer of the cell is labeled ectoplasm; the inner layer is labeled endoplasm. A star-shaped structure is labeled contractile vacuole.
Figure 2.47: a) A micrograph showing a circular dome with long branches emanating outward. B) A photograph showing a yellow structure that looks like foam on a branch.
Figure 2.48: A mature fruiting body produces a tall stalk with a sphere that generates spores via meiosis. The mature fruiting body releases spores. The haploid spores germinate and give rise to amoeba which divide to form more individual cells. Two amoeba fuse to form a zygote. The zygote can grow and undergo meiosis and multiple rounds of mitosis. The new haploid amoeba is released. Fertilization produces amoebas that aggregate into a structure called a slug. The slug migrates at a rate of 2 mm per hour. The migration stops the aggregate forms a fruiting body at the end of a stalk. This brings us back to the fruiting body in the life cycle.
Figure 2.49: A mature plasmodium (multinucleated free-flowing mass of protoplasm) can produce sclerotium (small cells) in a dry habitat. The mature plasmodium also produces diploid sporangia which produces haploid spores via meiosis. The mature sporangium releases mature spores which germinate. Germination gives rise to cells that can convert between ameboid and flagellated forms. Plasmogamy is the fusion of cytoplasm of two cells. Karyogamy is the fusion of nuclei and leads to the production of a diploid zygote. The zygote divides to form a multi-nucleated feeding plasmodium. This takes us back to the beginning of plasmodium stage of the life cycle.
Figure 2.50: a) A diagram of an apicomplexan protist. The cell is a long oval with an apical complex at the apical end. B) A micrograph of the protist showing a long oval.
Figure 2.51: A micrograph of an oval cell with many short projections.
Figure 2.52: Paramecium cell with short strands on the outside labeled cilia. An indent in the outer layer is labeled cytostome. The outside edge of the cytostome is an indent in the cell labeled oral groove. A sphere inside the cell at the base of the cytostome is labeled food vacuole, another nearby sphere is labeled cytoproct. A smaller opening in the cell is labeled anal pore. A star-shaped structure inside the cell is labeled contractile vacuole. A large oval is labeled macronucleus, and a smaller oval is labeled micronucleus.
Figure 2.53: A micrograph of long trumpet shaped cells. The wide part of the cell has an oval structure labeled cytostome and small projections labeled cilia.
Figure 2.54: A photograph of an insect covered in white fuzz labeled water mold.
Figure 2.55: An oval cell with a long flagellum at one end near the photoreceptor (paraflagellar body). A large oval inside the cell is labeled nucleus and contains a smaller oval labeled nucleolus. Green structures are labeled chloroplasts. A red circle is labeled stigma (eyespot). Another sphere is labeled contractile vacuole and a large sphere is labeled pellicle bands. Gray structures are labeled polysaccharides stored by photosynthesis.
Figure 2.56: The life cycle of Trypanosoma brucei takes place in both tsetse fly and humans. When the tsetse fly takes a blood meal it injects T. brucei into the bloodstream of a human. There the T. brucei multiplies by binary fission in blood, lymph, and spinal fluid. When another tsetse fly takes a blood meal it ingests T. brucei which multiplies by binary fission in the midgut of the fly. The T. brucei then transforms into an infectious stage which enters the salivary glands and multiplies. This can then be spread to another human.
Figure 2.57: A micrograph of a small clear tapered worm.
Figure 2.58: a) Class Turbellaria – a photograph of a flat oval-shaped worm labeled Pseudobiceros bedfordi. B) Class Monogenea – a micrograph of a rectangular cell with a bulb at one end. Labeled Dactylogyrus sp. C) Class Trematoda – A photograph of a long oval organism labeled Fascioloides magna and two smaller oval organisms labeled Fasciola hepatica. D) Class Cestoda – A photograph of a very long tapeworm labeled Taenia saginata.
Figure 2.59: a) A micrograph of Opisthorchis viverrini; an oval cell with a projection at one end. B) A micrograph of one end of Taenia solium showing a whorl of small projections above an area labeled scolex.
Figure 2.60: Schistosoma mansoni, japonicum, and haematobium are found in feces; S. japonicum and S. haematobium are also found in urine. These can be diagnosed in the water and produce eggs which hatch releasing miracidia. The miracidia penetrate snail tissues and produce sporocysts in the snail (successive generations). The Cercariae released by snail into the water are free flowing and are the infective stage which can penetrate skin. S. mansoni travels to the large intestines, S. japonicum travels to the small intestines, and S. haematobium travels to the rectum. The cercariae lose their tails during penetration and become schistosomula. These enter circulation and migrate to portal blood in liver and mature into adults. The paired adult worms migrate to the mesenteric venules of the bowels/rectum (laying eggs that circulate to the liver and are shed in stools) – for S. mansoni and S. Japonicum. S. haematobium migrates to the venous plexus of the bladder.
Figure 2.61: Eggs or gravid proglottids from an infected individual are passed into the environment; this is the diagnostic stage. Cattle (T. saginata) and pigs (T. solium) become infected by ingesting vegetation contaminated by eggs or gravid proglottids. Oncospheres hatch, penetrating intestinal wall and circulate to musculature. The oncospheres develop into cysticerci in muscles and become infective. Humans are infected by ingesting raw or undercooked infected meat. The scolex attaches to intestine and adults are found in the small intestine.
Figure 2.62: Molds can have septate hyphae – long strands with cell walls separating the nuclei. Or they can have coenocytic (nonseptate) hyphae – long strands with no cell wall separating the nuclei. Or they can have pseudohyphae which look like chains of cells with small clusters at intervals.
Figure 2.63: Drawing of bats in an attic. Fungal body is shown in the guano. A micrograph of the fungus shows hyphae (long strands) with spheres labeled conidia. The life cycle shows a person inhaling spores which then travel to the lungs and divide into a yeast form. They then travel to the lymph and blood.
Figure 2.64: Zygomycete life cycle. The mycelia can undergo asexual reproduction by forming spores via mitosis. The spores then form mycelia by germination. The haploid spores can also undergo sexual reproduction. The first step is germination when mycelia form. If the two mating types (+ and -) are in close proximity, extensions called gametangia form between them. Next is plasmogamy. This is the fusion between the + and – mating types, resulting in a zygosporangium with multiple haploid nuclei. The zygosporangium forms a thick, protective coat. Next the nuclei fuse to form a zygote with multiple diploid nuclei in karyogamy. This forms a diploid zygote. Next is mitosis and germination where the sporangium grows on a short stalk and the haploid spores are formed inside. The spores are released in germination and we are back to the spore stage of the life cycle.
Figure 2.65: a) A micrograph of long strands labeled hyphae and a sphere (labeled sporangium) on the end of one of the long strands. B) A photograph of bread mold. The white fuzz has black dots labeled sporangia.
Figure 2.66: a) a micrograph of a large oval (10 µm) labeled ascus and smaller ovals (5 µm) labeled ascospores. B) a micrograph of a long stalk with strands of spheres emanating from a sphere on the tip. The spheres are about 2 µm in diameter. C) A long strand with clusters of spheres. A small dot in each sphere is labeled nucleus.
Figure 2.67: A micrograph showing a thick tube with 8 ovals lined up within the tube.
Figure 2.68: Ascomycete life cycle. Mycelia produce conidiophores which use mitosis to asexually produce spores. These spores then germinate into new mycelia. Sexual reproduction begins one hyphae produces an ascogonium and another produces an antheridium. In plasmogamy the ascogonium and antheridium fuse. Mitosis and cell division result in the formation of many dikaryotic hyphae, which form a fruiting body called the ascocarp. Asci form at the tips of these hyphae. In karyogamy the nuclein in the asci fuse to form a diploid zygote. Then meiosis produces four haploid nuclei in the ascus. Then mitosis and cell division results in eight haploid ascospores in the ascus. These ascospores then disperse and germinate into new mycelia.
Figure 2.69: Basidiomycete life cycle. Haploid basidiospores germinate to form mycelia. There are two mating types (+ and -_). In plasmogamy, fusion between + and – mating types results in formation of a dikaryotic mycelium. Under the right environmental conditions, a basidiocarp forms via mitosis. Gills of the basidiocarp contain cells called basidia. A photo of a mushroom labels the mushroom as basidiocarp and basidia within the gills. Basidia form diploid nuclei via karyogamy; this produces a diploid zygote. Four haploid nuclei are formed in the basidium via meiosis. Cell division produces four haploid basidiospores. These spores then disperse and germinate into new mycelia.
Figure 2.70: Figure a is an electron micrograph showing a virus on the surface of a bacterial cell. The virus has a large head region, a thick neck and thin spider-like legs attached to the bacterium. Figure b is a drawing that labels the outside of the head as the capsid with the viral genome inside. The neck as the sheath and the legs as tail fibers.
Figure 2.71: A scale is showing sizes of various entities. The largest is a frog egg and 1 mm. Human egg cells and pollen are approximately 400 µm. Typical plant and animal cells range from 10 to 100 µm. Red blood cells are under 10 µm. Mitochondria and bacteria are approximately 1 µm. Smallpox is approximately 500 nm. Flu virus is approximately 100 nm. Polio virus is approximately 50 nm. Proteins range from 5 – 10 nm. Lipids range from 1 – 5 nm. Atoms are approximately 0.1 nm.
Figure 2.72: Part A shows a micrograph of atadenovirus, which looks like a wispy sphere that has a larger, flatter structure attached to the bottom. To the right of that is an illustration of the atadenovirus that labels capsomeres, capsids, DNA, and spikes made of glycoproteins. Part B shows the enveloped human immunodeficiency virus in black and white. To the right is an illustration that labels the matrix protein, viral envelope, spikes made of glycoproteins, reverse transcriptase, capsids, and RNA.
Figure 2.73: Figure a is a helical virus which has a long linear structure. The outer proteins are small spheres arranged into a long, hollow tube. Inside the tube is the genetic material. Tobacco mosaic virus is an example of a helical virus. Figure b is an Icosahedral viruses have a polyhedron structure. The example shown is human rhinovirus which has a pentagon structure. Complex viruses have a more complex structure. The example is variola which has an ovoid structure.
Figure 2.74: This figure outlines the stages of the lytic cycle. Step 1 is attachment when the phage attaches to the surface of the host. The bacteriophage is shown sitting on the surface of the bacterial host cell. Step 2 is penetration when the viral DNA enters the host cell. The image shows DNA from within the virus being injected into the host DNA. Step 3 is biosynthesis when the phage DNA replicates and the phage proteins are made. The image shows various pieces of virus being built within the cell. Step 4 is maturation when the new phage particles are assembled. This shows the viral components being put together in the cell. The fifth step is lysis when the cell lyses and the newly made phages are released. This shows the cell bursting and built viruses being released.
Figure 2.75: The steps of the lytic and lysogenic cycles. First the phage infects a cell; this shows the virus sitting on the outside of a cell and injecting DNA into the cell. In the next step the phage DNA becomes incorporated into the host genome. In the next step, the cell divides and prophage DNA is passed to the daughter cells. The image shows the cell dividing and the viral DNA within the host genome also being passed to the daughter cell. The next step shows the viral DNA jumping out of the host genome. Under stressful conditions, the prophage DNA is excised from the bacterial chromosomes and enters the lytic cycle. Next, the phage DNA replicates and phage proteins are made. This shows viral pieces being made within the cell. The next step is when the new phage particles are assembled. This shows the virus being build. The final step is when the cell lyses and releases the newly made phages.
Figure 2.76: The steps of specialized transduction. Step 1 is viral attachment and penetration. This is when the phage infects a cell. This shows the virus sitting on the outside of a cell and injecting DNA into the cell. Step 2 is integration when the phage DNA becomes incorporated into the host genome. Step 3 is excision when the phage is excised from the bacterial chromosomes along with a short piece of bacterial DNA. The DNA is then packaged into newly formed capsids. When the virus particles are assembled the DNA contains both viral and host segments. Step 4 is infection when the phage contains both viral and bacterial DNA infects a new host cell. Step 5 is recombination when the phage DNA along with the attached bacterial DNA are incorporated into a new cell. The image shows a new bacterial cell with virus DNA as well as other bacterial DNA in its genome.
Figure 2.77: Steps of influenza infection. Step 1 is attachment when the influenza virus becomes attached to a target epithelial cell. This image shows a spherical virus binding to the surface of a host cell. Step 2 is penetration when the cell engulfs the virus by endocytosis; this shows the virus within a vacuole. Step 3 is uncoating when the viral contents are released; the image shows the virus being released from the vacuole. Step 4 is biosynthesis when the viral RNA enters the nucleus where it is replicated by RNA polymerase. Step 5 is assembly when the new phage particles are assembled. Step 6: release when new viral particles are made and released into the extracellular fluid. The cell, which is not killed in the process continues to make new viruses.
Figure 2.78: Viruses with −ssRNA (negative single-stranded RNA) use RdRP (viral RNA-dependent RNA polymerase) to make +ssRNA (positive single-stranded RNA). RdRP can also be used to convert +ssRNA to −ssRNA. +ssRNA uses host ribosomes to make viral proteins.
Figure 2.79: The HIV viral cycle. Step 1: the HIV fuses to the host-cell surface. Specifically, the gp120 proteins on the surface of the virus binds to the CD4. This then binds to a smaller coreceptor (CCR5 or CXCR4). Step 2: HIV RNA, reverse transcriptase, integrase, and other viral proteins enter the host cell. The virus is brought into the host cell and uncoated; both viral RNA and reverse transcriptase are loosed into the cell. Step 3: Viral DNA is formed by reverse transcription. Step 4: Viral DNA is transported across the nucleus and integrates into the host DNA. Integrase found on the viral DNA. The viral DNA in the host DNA is called provirus. Step 5: New viral RNA is used as genomic RNA and to make viral proteins. New viral RNA strands are made and leave the nucleus. Step 6: New viral RNA and proteins move to the cell surface and a new immature HIV forms. The viral is assembled in an out bulging of the host cell. Step 7: The virus matures when protease releases the proteins that form the mature HIV. Mature virion is released.
Figure 2.80: Figure A is an electron micrograph that shows a sphere within a larger blob-shaped structure. Figure B shows raised red dots on a person’s face.
Figure 2.81: The Y axis of the graph is “logarithm of number of infectious virions”; the X axis of the graph is “hours”. The beginning of the line has a downward slope and is labeled “1: Inoculation: inoculum of virus binds to cells”. Next is a flat region of the line labeled “2: Eclipse: virions penetrate the cell”. Next is an upward slope labeled “3: Burst: host cells release many viral particles”. Next is another flat region labeled “4: Burst size: number of virions released per bacterium”.
Figure 2.82: This chart has three columns labeled components, interactions, and microtiter results. In row A the components are the red blood cells which do not interact with anything and show no reaction in a microtiter result. The lack of reaction is seen as a small red dot in the center of the well. In row B the components are viruses and red blood cells. The viruses and red blood cells clump together and this is seen in a microtiter result as redness throughout the well. This is called hemagglutination. In row C, the components are viruses, red blood cells and antibodies. The viruses and antibodies clump together but the red blood cells do not clump with anything. This is again seen as no reaction; this is called hemagglutination inhibition.
Figure 2.83: The explanation of EIA is separated to show what occurs in a positive sample and what occurs in a negative sample. First patient sample is applied to a membrane filter. If the sample contains viruses they are trapped by the filter. Next, antibody with enzyme conjugate is added. Antibody will attach to antigen if present. Next is a wash step. If the virus is present the enzyme binds to the virus, otherwise the enzyme washes away. Finally substrate is added. If the antibody is present (because it is bound to the virus) the attached enzyme causes a color change. If no enzyme linked antibody is present, no color change occurs.
Figure 2.84: Figure A shows the process of how normal prion protein is converted to disease causing forms. Endogenous PrPc interact with PrpSC. This converts PRPC into PRPSC. The PRPSC accumulate. Figure B is a micrograph that shows holes in brain tissue.
Figure 2.85: The CJD brain has larger spaces as seen by more black regions in the image of the whole brain. The micrograph shows holes in the brain tissue.
Figure 2.86: A diagram Koch’s postulates. 1 – The suspected causative agent must be absent from all healthy organisms but present in all diseased organisms. This is demonstrated by looking at slides under a microscope from a sick mouse and seeing the suspected agent. A slide from a healthy mouse only shows healthy red blood cells. 2 – The causative agent must be isolated from the diseased organism and grown in pure culture. This is demonstrated by showing growth on a petri plate from the sick mouse and no growth from the healthy mouse. 3 – The cultured agent must cause the same disease when inoculated into a healthy, susceptible organism. This is demonstrated by injecting a healthy mouse with the cultured agent and having that mouse get sick. 4 – The same causative agent must then be reisolated from the inoculated diseased organism. This is demonstrated by a Petri plate from this last mouse showing the growth of the causative agent.
Figure 2.87: A graph with “number of pathogenic agents (cells or virions)” on the X axis and Percent mortality in experimental group on the Y axis. The graph begins at 0,0 and increases until there is nearly 100% death at 10 to the 5. The line then plateaus at 100%. A 50% death rate occurs at 10 to the 4. This is the LD 50.
Figure 2.88: Portals of entry: eye (conjunctiva), nose, mouth, ear, needle, broken skin, insect bite, urethra, vagina, anus, placenta (portal of entry for fetus).
Figure 2.89: Micrograph of round cells attached to a surface by long strands.
Figure 2.90: Diagram of H. pylori invading the lining of the stomach. In the first image the H. pylori (an oval cell with 3 flagella is not able to penetrate the gastric mucin gel on top of the epithelial cells. Contact with stomach acid keeps the mucin lining the epithelial cell layer in a spongy gel-like state. This consistency is impermeable to the bacterium H. pylori. The second image shows the bacterium entering the lining. The bacterium releases urease, which neutralizes the stomach acid. This causes the mucin to liquefy and the bacterium can swim right through it.
Figure 2.91: Portals of exit: eye (tears), needle, mammary glands (milk, secretions), placenta (transmission to fetus), vagina (secretions, blood), urethra (urine), broken skin, broken skin (blood), skin (flakes), nose (secretions), mouth (saliva, sputum), ear (earwax), urethra (urine, semen, secretions), anus (feces).
Figure 2.92: A picture of a person with a swollen right hand.
Figure 2.93: a) A diagram of epithelial cells that are connected along their membranes. Hyaluronidases enter at these connection points. B) after the hyaluronidases break down the connections between the cells, bacteria can flow through the openings.
Figure 2.94: A long chain of O antigens is drawn as various geometric shapes in a long row. Next is a core; a shorter region of similar shapes. Next is 2 circles labeled lipid A. Each of these has 2 or 3 long wavy lines projecting from them.
Figure 2.95: Diagram of how the A-B toxin works. The B subunit binds to a cellular receptor on the cell membrane. The A subunit is bound to the B subunit at this point. The cell engulfs the toxins into a vacuole. Inside the vacuole, which is acidic, the a subunit dissociates and escapes into the cytoplasm.
Figure 2.96: A diagram of the mechanism of diphtheria toxin. On the outside is a membrane with the B subunit attached. Inside is the A subunit binding with NAD. This block EF-2 by binding ADP-ribose. The diagram also shows mRNA bound to a ribosome and protein being made. The A subunit causes elongation of the protein to stop.
Figure 2.97: Botulinum toxin causes flaccid paralysis and stops muscle contraction. The diagram shows that the normal mechanism has acetylcholine released at the axon terminal. The acetylcholine then binds to receptors on the membrane of a muscle cell. The abnormal mechanism – botulinum toxin blocks the release of acetylcholine stopping muscle contraction. Tetanus toxin causes spastic paralysis; uncontrollable muscle contraction. The normal mechanism shows glycine and GABA released from one axon terminal and binding to receptors on another axon terminal. This causes acetylcholine to remain in the vesicles and not bind to the receptors on the muscle cell. In the abnormal mechanism tetanus toxin prevents the release of glycine and GABA. Therefore acetylcholine is released and binds to receptors on the muscle. This prevents the relaxation of muscles.
Figure 2.98: A) a micrograph showing nonencapsulated cells as blue ovals on a light background. Encapsulated cells have a thick clear ring around the blue cells. B) Antibodies on phagocytic cells bind to antigens on the bacterial cell. Capsules on the bacterial cell cover the antigen and prevent the antibody from binding to the antigen. C) A bacterial cell is releasing small dots labeled proteases that are breaking down an antibody.
Figure 2.99: A) antigenic drift results from genetic mutations. Virus A is shown with different shaped pieces on the outside labeled neuraminidase and hemagglutinin. The mutated hemagglutinin has a different shape. B) Antigenic shift results from genetic reassortment. Virus A has green hemagglutinin and orange neuraminidase on the outside. Virus B has purple neuraminidase and blue hemagglutinin. These both enter the same host cell. Virus C is then produced which has the neuraminidase from virus A and the hemagglutinin from virus B.
Figure References
Figure 2.1: A typical prokaryotic cell contains a cell membrane, chromosomal DNA that is concentrated in a nucleoid, ribosomes, and a cell wall. (c) Rice University. OpenStax Microbiology. CC BY 4.0. https://openstax.org/details/books/microbiology.
Table 2.1: Common prokaryotic cell shapes. Illustrations: (c) Rice University. OpenStax Microbiology. CC BY 4.0. https://openstax.org/details/books/microbiology. Coccus micrograph: modification of work by Janice Haney Carr, Centers for Disease Control and Prevention. Public Domain. Bacillus micrograph: modification of work by National Institute of Allery and Infectious Diseases. Public Domain. https://commons.wikimedia.org/wiki/File:E._coli_Bacteria_(7316101966).jpg. Vibrio micrograph: modification of work by Janice Haney Carr, Centers for Disease Control and Prevention. Public Domain. https://phil.cdc.gov/Details.aspx?pid=6937. Coccobacillus micrograph: modification of work by Janice Carr, Centers for Disease Control and Prevention. Public Domain. Spirillum micrograph: modification of work by Wolframm Adlassnig. Public Domain. https://commons.wikimedia.org/wiki/File:Spirillen.jpg Spirochete micrograph: modification of work by Centers for Disease Control and Prevention. Public Domain.
Table 2.2: Common prokaryotic cell arrangements. (c) Rice University. OpenStax Microbiology. CC BY 4.0. https://openstax.org/details/books/microbiology.
Figure 2.2: The nucleoid region (the area enclosed by the green dashed line) is a condensed area of DNA found within prokaryotic cells. (c) Rice University. OpenStax Microbiology. CC BY 4.0. https://openstax.org/details/books/microbiology.
Figure 2.3: There are three prokaryote-specific mechanisms leading to horizontal gene transfer in prokaryotes. (c) Rice University. OpenStax Microbiology. CC BY 4.0. https://openstax.org/details/books/microbiology.
Figure 2.4: Prokaryotic ribosomes (70S) are composed of two subunits: the 30S (small subunit) and the 50S (large subunit), each of which are composed of protein and rRNA components. (c) Rice University. OpenStax Microbiology. CC BY 4.0. https://openstax.org/details/books/microbiology.
Figure 2.5: Prokaryotic cells may have various types of inclusions. Image a. Figure 5A in Moore, R.A., Tuanyok, A. & Woods, D.E. Survival of Burkholderia pseudomallei in Water. BMC Res Notes 1, 11 (2008). https://doi.org/10.1186/1756-0500-1-11. CC BY 2.0, Images b, c, d: (c) American Society of Microbiology. Redistribution authorized with attribution. Image e: Modified from Figure 3 in Blondeau, M., Guyodo, Y., Guyot, F. et al. Magnetic-field induced rotation of magnetosome chains in silicified magnetotactic bacteria. Sci Rep 8, 7699 (2018). https://doi.org/10.1038/s41598-018-25972-x. CC BY 4.0. Images b, c, d: (c) American Society of Microbiology. Redistribution authorized with attribution.
Figure 2.6: Sporulation begins following asymmetric cell division. Image a: (c) Rice University. OpenStax Microbiology. CC BY 4.0. https://openstax.org/details/books/microbiology. Image b: (c) Jonathan Eisen. CC BY 1.0 Generic. https://commons.wikimedia.org/wiki/File:Electron_micrograph_of_endospore_of_the_bacterium_Carboxydothermus_hydrogenoformans.png. Image c: Centers for Disease Control and Prevention. Public Domain. https://phil.cdc.gov/Details.aspx?pid=1894
Figure 2.7: Peptidoglycan is composed of polymers of alternating NAM and NAG subunits, which are cross-linked by peptide bridges linking NAM subunits from various glycan chains. (c) Rice University. OpenStax Microbiology. CC BY 4.0. https://openstax.org/details/books/microbiology.
Figure 2.8: Bacteria contain two common cell wall structural types. (c) Rice University. CC BY 4.0. Modification of work (c) Franciscosp2 “Bacteria cell wall2”. CC BY 3.0 Unported. https://commons.wikimedia.org/wiki/File:Bacteria_cell_wall2.svg
Figure 2.9: Some gram-positive bacteria, including members of the Mycobacteriaceae, produce waxy mycolic acids found exterior to their structurally-distinct peptidoglycan. Left: (c) Rice University CC BY 4.0. Modification of work by (c) Franciscosp2 “Bacteria cell wall2”. CC BY 3.0 Unported. https://commons.wikimedia.org/wiki/File:Bacteria_cell_wall2.svg. Right: Modification of work by Centers for Disease Control and Prevention/Dr George P. Kobica. Public Domain. https://commons.wikimedia.org/wiki/File:Mycobacterium_tuberculosis_Ziehl-Neelsen_stain_02.jpg
Figure 2.10: The outer membrane of a gram-negative bacterial cell contains lipopolysaccharide (LPS), a toxin composed of Lipid A embedded in the outer membrane, a core polysaccharide, and the O-side chain. (c) Rice University. OpenStax Microbiology. CC BY 4.0. https://openstax.org/details/books/microbiology.
Figure 2.11: Capsules are a type of glycocalyx composed of an organized layer of polysaccharides. Left: (c) Rice University. OpenStax Microbiology. CC BY 4.0. https://openstax.org/details/books/microbiology. Right: (c) American Society of Microbiology. Redistribution authorized with attribution.
Figure 2.12: Bacteria may produce two different types of protein appendages that aid in surface attachment. (c) American Society of Microbiology. Redistribution authorized with attribution.
Figure 2.13: The basic structure of a bacterial flagellum consists of a basal body, hook, and filament. Modification of work by LadyofHats/Mariana Ruiz Villareal. Public Domain. https://commons.wikimedia.org/wiki/File:Flagellum_base_diagram-en.svg
Figure 2.14: Flagellated bacteria may exhibit multiple arrangements of their flagella. (c) Rice University. OpenStax Microbiology. CC BY 4.0. https://openstax.org/details/books/microbiology.
Figure 2.15: Bacteria achieve directional movement by changing the rotation of their flagella. (c) Rice University. OpenStax Microbiology. CC BY 4.0. https://openstax.org/details/books/microbiology.
Table 2.5: Gram staining process. Gram-staining is a differential staining technique that uses a primary stain and a secondary counterstain to distinguish between gram-positive and gram-negative bacteria. (c) Rice University. OpenStax Microbiology. CC BY 4.0. https://openstax.org/details/books/microbiology.
Figure 2.16: In this specimen, the gram-positive bacterium Staphylococcus aureus retains crystal violet dye even after the decolorizing agent is added. Modification of work (c) Nina Parker. CC BY 4.0.
Figure 2.17: Ziehl-Neelsen staining has rendered these Mycobacterium tuberculosis cells red and the surrounding growth indicator methylene blue. (c) American Society of Microbiology. Redistribution authorized with attribution.
Figure 2.18: India-ink was used to stain the background around these cells of the yeast Cryptococcus neoformans. (c) American Society of Microbiology. Redistribution authorized with attribution.
Figure 2.19: A stained preparation of Bacillus subtilis showing endospores as green and the vegetative cells as pink. (c) American Society of Microbiology. Redistribution authorized with attribution.
Figure 2.20: A flagella stain of Bacillus cereus, a common cause of foodborne illness, reveals that the cells have numerous flagella used for locomotion. Modification of work by Centers for Disease Control and Prevention. Public Domain.
Table 2.6: Simple stains. Top: Modification of work by Centers for Disease Control and Prevention. Public Domain. Middle: Modification of Figure 1C in Danovaro, R., Dell’Anno, A., Pusceddu, A. et al. The first metazoa living in permanently anoxic conditions. BMC Biol 8, 30 (2010). https://doi.org/10.1186/1741-7007-8-30. CC BY 2.0. Bottom: (c) modification of work by Anh-Hue Tu. CC BY 4.0.
Figure 2.21: The electron micrograph depicts two cells of Salmonella typhimurium after a binary fission event. Left: modification of work by Centers for Disease Control and Prevention. Public Domain. Right: (c) Rice University. OpenStax Microbiology. CC BY 4.0. https://openstax.org/details/books/microbiology.
Figure 2.22: The growth curve of a bacterial culture is represented by the logarithm of the number of live cells plotted as a function of time. (c) Rice University. OpenStax Microbiology. CC BY 4.0. https://openstax.org/details/books/microbiology.
Figure 2.23: Both graphs illustrate population growth during the log phase for a bacterial sample with an initial population of one cell and a doubling time of 1 hour. (c) Rice University. OpenStax Microbiology. CC BY 4.0. https://openstax.org/details/books/microbiology.
Figure 2.24: Rickettsias require special staining methods to see them under a microscope. modification of work by Centers for Disease Control and Prevention. Public Domain.
Figure 2.25: Neisseria meningitidis growing in colonies on a chocolate agar plate. Centers for Disease Control and Prevention. Public Domain.
Figure 2.26: Aliivibrio fischeri is a bioluminescent bacterium. Left: (c) American Society of Microbiology. Redistribution authorized with attribution. Right: modification of work (c) Margaret McFall-Ngai. CC BY 4.0. https://commons.wikimedia.org/wiki/File:Hawaiian_Bobtail_squid.tiff
Figure 2.27: Legionella pneumophila, the causative agent of Legionnaires disease, thrives in warm water. Left: Modification of work by Centers for Disease Control and Prevention. Public Domain. Right: Public Domain.
Figure 2.28: Salmonella typhi is the causative agent of typhoid fever. Centers for Disease Control and Prevention. Public Domain.
Figure 2.29: Myxobacteria form fruiting bodies. Modification of work by Michiel Vos in (2005) Antisocial Behavior in Cooperative Bacteria (or, Why Can’t Bacteria Just Get Along?). PLoS Biol 3(11): e398. https://doi.org/10.1371/journal.pbio.0030398. https://commons.wikimedia.org/wiki/File:Myxococcus_xanthus.png. CC BY 2.5.
Figure 2.30: Helicobacter pylori can cause chronic gastritis, which can lead to ulcers and stomach cancer. By Yutaka Tsutsumi. Permission granted. https://commons.wikimedia.org/wiki/File:EMpylori.jpg
Figure 2.31: Chlamydia begins infection of a host when the metabolically inactive elementary bodies enter an epithelial cell. modification of work by Centers for Disease Control and Prevention. Public Domain.
Figure 2.32: Spirochetes are typically observed using darkfield microscopy (left). Left: modification of work by Centers for Disease Control and Prevention. Public Domain. Middle: (c) modification of Figure 2a and e by Guyard C, Raffel SJ, Schrumpf ME, Dahlstrom E, Sturdevant D, Ricklefs SM, et al. (2013) Periplasmic Flagellar Export Apparatus Protein, FliH, Is Involved in Post-Transcriptional Regulation of FlaB, Motility and Virulence of the Relapsing Fever Spirochete Borrelia hermsii. PLoS ONE 8(8): e72550. https://doi.org/10.1371/journal.pone.0072550. CC0/Public Domain. Right: (c) Rice University. OpenStax Microbiology. CC BY 4.0. https://openstax.org/details/books/microbiology
Figure 2.33: Bacteroides comprise up to 30% of the normal microbiota in the human gut. NOAA. Public domain.
Figure 2.34: (a) Sessile Planctomycetes have a holdfast that allows them to adhere to surfaces in aquatic environments. (c) American Society of Microbiology. Redistribution authorized with attribution.
Figure 2.35: Purple and green sulfur bacteria use bacteriochlorophylls to perform photosynthesis. Modification of work by kOchstudiO. Public Domain. https://commons.wikimedia.org/wiki/File:Green_d_winogradsky.jpg
Figure 2.36: (a) Microcystis aeruginosa is a type of cyanobacteria commonly found in freshwater environments. Credit a: modification of work by Dr. Barry H. Rosen/U.S. Geological Survey; credit b: modification of work by NOAA. Public domain.
Figure 2.37: Actinomyces israelii. Credit a: Modification of work by Graham Beards. CC BY 3.0 https://commons.wikimedia.org/wiki/File:Actinomyces_israelii.jpg. Credit b: modification of work by Centers for Disease Control and Prevention. Public Domain https://commons.wikimedia.org/wiki/File:Corynebacterium_diphtheriae_Gram_stain.jpg. Credit c: modification of Figure 3 in Mwakigonja, A.R., Torres, L.M.M., Mwakyoma, H.A. et al. Cervical cytological changes in HIV-infected patients attending care and treatment clinic at Muhimbili National Hospital, Dar es Salaam, Tanzania. Infect Agents Cancer 7, 3 (2012). https://doi.org/10.1186/1750-9378-7-3. CC BY 2.0.
Figure 2.38: Clostridium difficile, a gram-positive, rod-shaped bacterium, causes severe colitis and diarrhea, often after the normal gut microbiota is eradicated by antibiotics. modification of work by Centers for Disease Control and Prevention. Public Domain.
Figure 2.39: A gram-stained specimen of Streptococcus pyogenes shows the chains of cocci characteristic of this organism’s morphology. (c) American Society of Microbiology. Redistribution authorized with attribution.
Figure 2.40: In this gram-stained specimen, the violet rod-shaped cells forming chains are the gram-positive bacteria Bacillus cereus. Credit a: modification of work by (c) Bibliomaniac 15. CC BY 3.0 Unported. https://commons.wikimedia.org/wiki/File:Bacillus_cereus_and_Escherichia_coli.jpg; credit b: modification of work by Centers for Disease Control and Prevention. Public Domain. https://commons.wikimedia.org/wiki/File:Bacillus_cereus_01.png
Figure 2.41: This SEM of Staphylococcus aureus illustrates the typical grape-like clustering of cells. modification of work by Centers for Disease Control and Prevention. Public Domain.
Figure 2.42: M. tuberculosis grows on Löwenstein-Jensen (LJ) agar in distinct colonies. Centers for Disease Control and Prevention. Public Domain.
Figure 2.43: Ringworm presents as a raised ring, which is gray or brown on brown or black skin (a), and red on lighter skin (b). Centers for Disease Control and Prevention. Public Domain.
Figure 2.44 A scanning electron micrograph shows many Giardia parasites in the trophozoite, or feeding stage, in a gerbil intestine. modification of work by Centers for Disease Control and Prevention. Public Domain.
Figure 2.45: In the sexual/asexual life cycle of Eimeria, oocysts (inset) are shed in feces and may cause disease when ingested by a new host. “life cycle,” “micrograph”: modification of work by USDA. Public domain.
Figure 2.46: Paramecium spp. have hair-like appendages called cilia for locomotion. (c) Rice University. OpenStax Microbiology. CC BY 4.0. https://openstax.org/details/books/microbiology.
Figure 2.47: The cellular slime mold Dictyostelium discoideum. By Bruno in Columbus. Public Domain. https://commons.wikimedia.org/wiki/File:Dictyostelium_Aggregation.JPG. (b) Fuligo septica. By Siga. Public Domain. https://commons.wikimedia.org/wiki/File:Fuligo_septica_bl1.JPG
Figure 2.48: The life cycle of the cellular slime mold Dictyostelium discoideum. (c) Rice University. OpenStax Microbiology. CC BY 4.0. https://openstax.org/details/books/microbiology. Modification of photo (c) thatredhead4. CC BY 2.0. https://flic.kr/p/6JdBCD
Figure 2.49: Plasmodial slime molds exist as large multinucleate amoeboid cells that form reproductive stalks to produce spores that divide into gametes. (c) Rice University. OpenStax Microbiology. CC BY 4.0. https://openstax.org/details/books/microbiology.
Figure 2.50: Apicomplexans are parasitic protists. Left: (c) Rice University. OpenStax Microbiology. CC BY 4.0. https://openstax.org/details/books/microbiology. Right: Modification of work by (c) Ute Frevert. CC BY 42.5 Generic. https://commons.wikimedia.org/wiki/File:Malaria.jpg
Figure 2.51: This specimen of the ciliate Balantidium coli is a trophozoite form isolated from the gut of a primate. modification of Figure 2g in Kouassi RY, McGraw SW, Yao PK, Abou-Bacar A, Brunet J, Pesson B, Bonfoh B, N’goran EK, Candolfi E. Diversity and prevalence of gastrointestinal parasites in seven non-human primates of the Taï National Park, Côte d’Ivoire. Parasite. 2015;22:1. doi: 10.1051/parasite/2015001. CC BY 4.0. https://commons.wikimedia.org/wiki/File:Balantidium_coli_trophozoite.jpg
Figure 2.52: Paramecium has a primitive mouth (called an oral groove) to ingest food, and an anal pore to excrete it. Left: (c) Rice University. OpenStax Microbiology. CC BY 4.0. https://openstax.org/details/books/microbiology. Right: Luis Fernández García. CC BY SA 3.0 Unported. https://commons.wikimedia.org/wiki/File:Paramecium.jpg
Figure 2.53: This differential interference contrast micrograph (magnification: ×65) of Stentor roeselie shows cilia present on the margins of the structure surrounding the cytostome; the cilia move food particles. Modification of work (c) picturepest. https://flic.kr/p/oRgy8Q. CC BY 2.0.
Figure 2.54: A saprobic oomycete, or water mold, engulfs a dead insect. Modification of work (c) Thomas Bresson. https://commons.wikimedia.org/wiki/File:Dead_insect_(6).jpg. CC BY 2.0.
Figure 2.55: This illustration of a Euglena shows the characteristic structures, such as the stigma and flagellum. Left: Modification of work (c) Claudio Miklos. Public Domain. https://commons.wikimedia.org/wiki/File:Euglena_diagram.jpg. Right: Modification of work by David Shykind. Public Domain. https://commons.wikimedia.org/wiki/File:Euglena_pellicle_2.jpg
Figure 2.56: Trypanosoma brucei, the causative agent of African trypanosomiasis, spends part of its life cycle in the tsetse fly and part in humans. modification of work by Centers for Disease Control and Prevention; credit “photo”: DPDx/Centers for Disease Control and Prevention. Public Domain.
Figure 2.57: A micrograph of the nematode Enterobius vermicularis, also known as the pinworm. modification of work by Centers for Disease Control and Prevention. Public Domain.
Figure 2.58: Phylum Platyhelminthes is divided into four classes. Images a, b, d. Public Domain. Image c: Modification of work (c) Flukeman CC BY 2.5 Generic. https://commons.wikimedia.org/wiki/File:F.magna_1_a_F.hepatica_2.jpg
Figure 2.59: The oral sucker is visible on the anterior end of this liver fluke, Opisthorchis viverrini. Left (c) modification of Figure 2 in Sripa B., Kaewkes S., Sithithaworn P., Mairiang E., Laha T., et al. (2007). “Liver Fluke Induces Cholangiocarcinoma”. PLoS Medicine 4(7): e201. doi:10.1371/journal.pmed.0040201. CC BY 2.5 Generic. https://commons.wikimedia.org/wiki/File:Opisthorchis_viverrini_3.png. Right: modification of work by Centers for Disease Control and Prevention. Public Domain.
Figure 2.60: The life cycle of Schistosoma spp. includes several species of water snails, which serve as secondary hosts. Illustration (c) Rice University. OpenStax Microbiology. CC BY 4.0. https://openstax.org/details/books/microbiology. Modification of work by Centers for Disease Control and Prevention. Public Domain. https://www.cdc.gov/dpdx/schistosomiasis/modules/Schistomes_LifeCycle_lg.jpg. Step 3 photo: modification of Figure 1 in (c) Lewis FA, Liang Y-s, Raghavan N, Knight M (2008) The NIH-NIAID Schistosomiasis Resource Center. PLoS Negl Trop Dis 2(7): e267. https://doi.org/10.1371/journal.pntd.0000267. CC BY.
Figure 2.61: Life cycle of a tapeworm. Illustration: Modification of work by Centers for Disease Control and Prevention. Step 1, 2, 4, 5, 6 Images: Public Domain. Step 3 Images: (c) American Society of Microbiology. Redistribution authorized with attribution.
Figure 2.62: Multicellular fungi (molds) form hyphae, which may be septate or nonseptate. (c) Rice University. OpenStax Microbiology. CC BY 4.0. https://openstax.org/details/books/microbiology.
Figure 2.63: Histoplasma capsulatum is a dimorphic fungus that grows in soil exposed to bird feces or bat feces (guano) (top left). modification of work by Centers for Disease Control and Prevention. Public Domain.
Figure 2.64: Zygomycetes have sexual and asexual life cycles. In the sexual life cycle, + and – mating types conjugate to form a zygosporangium. (c) Rice University. OpenStax Microbiology. CC BY 4.0. https://openstax.org/details/books/microbiology.
Figure 2.65: These images show asexually produced spores. Left: modification of work by Centers for Disease Control and Prevention. Public Domain. Right: modification of work by Ciar. Public Domain. https://commons.wikimedia.org/wiki/File:Moldy_old_bread.JPG
Figure 2.66: This brightfield micrograph shows ascospores being released from asci in the fungus Talaromyces flavus var. flavus. modification of work by Centers for Disease Control and Prevention. Public Domain.
Figure 2.67: These ascospores, lined up within an ascus, are produced sexually. (c) Peter G. Werner. CC BY 3.0 Unported. https://commons.wikimedia.org/wiki/File:Morelasci.jpg
Figure 2.68: The life cycle of an ascomycete is characterized by the production of asci during the sexual phase. (c) Rice University. OpenStax Microbiology. CC BY 4.0. https://openstax.org/details/books/microbiology.
Figure 2.69: The life cycle of a basidiomycete alternates a haploid generation with a prolonged stage in which two nuclei (dikaryon) are present in the hyphae. (c) Rice University. OpenStax Microbiology. CC BY 4.0. https://openstax.org/details/books/microbiology.
Table 2.17: Select groups of fungi. Image a: Ascomycota micrograph. modification of work by Dr. Lucille Georg, Centers for Disease Control and Prevention. Public Domain. https://commons.wikimedia.org/wiki/File:Aspergillus_niger_01.jpg. Image b: Basidiomycota. modification of work by Sanislaw Skowron. Public Domain. https://commons.wikimedia.org/wiki/File:Amanita_phalloides_young.jpg. Image c: Microsporidia micrograph: modification of work by Centers for Disease Control and Prevention. Public Domain. https://www.cdc.gov/dpdx/microsporidiosis/images/2/Microsporidia_2012.jpg. Image d: Zygomycota micrographs. Modification of work by Agong. Public Domain. https://commons.wikimedia.org/wiki/File:Rhizopus_fungus.jpg
Figure 2.70: (a) In this transmission electron micrograph, a bacteriophage (a virus that infects bacteria) is dwarfed by the bacterial cell it infects. Left: modification of work by U.S. Department of Energy, Office of Science, LBL, PBD. Public domain. Right: (c) Rice University. OpenStax Microbiology. CC BY 4.0. https://openstax.org/details/books/microbiology.
Figure 2.71: The size of a virus is small relative to the size of most bacterial and eukaryotic cells and their organelles. (c) Rice University. OpenStax Microbiology. CC BY 4.0. https://openstax.org/details/books/microbiology.
Figure 2.72: The naked atadenovirus uses spikes made of glycoproteins from its capsid to bind to host cells. Top Left: modification of work by NIAID. Public Domain. Bottom Left: modification of work by Centers for Disease Control and Prevention. Public Domain. Right: (c) Rice University. OpenStax Microbiology. CC BY 4.0. https://openstax.org/details/books/microbiology.
Figure 2.73: Viral capsids can be (a) helical, (b) polyhedral, or (c) have a complex shape. Top Left: modification of work by USDA ARS. Public Domain. Top Middle: Modification of work by U.S. Department of Energy. Public Domain. Top Right: University of Wisconsin-La Crosse, Microbiology program. Fair Use. https://web.archive.org/web/20220531010814/https://commons.wikimedia.org/wiki/File:Monkeypox.gif. Bottom: (c) Rice University. OpenStax Microbiology. CC BY 4.0. https://openstax.org/details/books/microbiology
Figure 2.74: A virulent phage shows only the lytic cycle pictured here. (c) Rice University. OpenStax Microbiology. CC BY 4.0. https://openstax.org/details/books/microbiology.
Figure 2.75: A temperate bacteriophage has both lytic and lysogenic cycles. (c) Rice University. OpenStax Microbiology. CC BY 4.0. https://openstax.org/details/books/microbiology.
Figure 2.76: This flowchart illustrates the mechanism of specialized transduction. (c) Rice University. OpenStax Microbiology. CC BY 4.0. https://openstax.org/details/books/microbiology.
Figure 2.77: Influenza virus is one of the few RNA viruses that replicates in the nucleus of cells. (c) Rice University. OpenStax Microbiology. CC BY 4.0. https://openstax.org/details/books/microbiology.
Figure 2.78: RNA viruses can contain +ssRNA that can be directly read by the ribosomes to synthesize viral proteins. (c) Rice University. OpenStax Microbiology. CC BY 4.0. https://openstax.org/details/books/microbiology.
Figure 2.79: HIV, an enveloped, icosahedral retrovirus, attaches to a cell surface receptor of an immune cell and fuses with the cell membrane. Modification of work by NIAID, NIH. Public domain.
Figure 2.80: Varicella-zoster, the virus that causes chickenpox. Left: Modification of work by Erskine Palmer and B.G. Partin/CDC. https://commons.wikimedia.org/wiki/File:Varicella_(Chickenpox)_Virus_PHIL_1878_lores.jpg. Scale-bar data from Matt Russell. CC BY 4.0. Right: (c) Paulo O. Fair Use. https://flic.kr/p/8Dt2fc
Figure 2.81: The one-step multiplication curve for a bacteriophage population follows three steps. (c) Rice University. OpenStax Microbiology. CC BY 4.0. https://openstax.org/details/books/microbiology.
Table 2.20: Cytopathic effects of specific viruses. (c) American Society of Microbiology. Redistribution authorized with attribution.
Table 2.20: Cytopathic effects of specific viruses. (c) American Society of Microbiology. Redistribution authorized with attribution.
Figure 2.82: This figure shows the possible outcomes of a hemagglutination test. modification of work by Centers for Disease Control and Prevention. Public Domain.
Figure 2.83: Similar to rapid, over-the-counter pregnancy tests, EIAs for viral antigens require a few drops of diluted patient serum or plasma applied to a membrane filter (1). Modification of work by “Cavitri. CC BY 3.0 Unported. https://commons.wikimedia.org/wiki/File:ELISA_diagram.png
Figure 2.84: Endogenous normal prion protein (PrPc) is converted into the disease-causing form (PrPsc) when it encounters this variant form of the protein. Left: (c) Rice University. OpenStax Microbiology. CC BY 4.0. https://openstax.org/details/books/microbiology. Right: Modification of work by USDA/Dr. Al Jenny. Public Domain. https://commons.wikimedia.org/wiki/File:Histology_bse.jpg
Figure 2.85: Creutzfeldt-Jakob disease (CJD) is a fatal disease that causes degeneration of neural tissue. Left: modification of work (c) Dr. Laughlin Dawes CC BY 4.0. Top Right: Modification of work (c) Suzanne Wakim. CC BY 4.0. Bottom right: modification of work by Centers for Disease Control and Prevention. Public Domain.
Figure 2.86: The steps for confirming that a pathogen is the cause of a particular disease using Koch’s postulates. (c) Rice University. OpenStax Microbiology. CC BY 4.0. https://openstax.org/details/books/microbiology.
Figure 2.87: A graph like this is used to determine LD50 by plotting pathogen concentration against the percent of infected test animals that have died. (c) Rice University. OpenStax Microbiology. CC BY 4.0. https://openstax.org/details/books/microbiology.
Figure 2.88: Shown are different portals of entry where pathogens can gain access into the body. (c) Rice University. OpenStax Microbiology. CC BY 4.0. https://openstax.org/details/books/microbiology.
Figure 2.89: Glycocalyx produced by bacteria in a biofilm allows the cells to adhere to host tissues and to medical devices such as the catheter surface shown here. Modification of work by Centers for Disease Control and Prevention. Public Domain.
Figure 2.90: H. pylori is able to invade the lining of the stomach by producing virulence factors that enable it to pass through the mucin layer covering epithelial cells. Modification of work by Zina Deretsky, National Science Foundation. Public domain.
Figure 2.91: Pathogens leave the body of an infected host through various portals of exit to infect new hosts. (c) Rice University. OpenStax Microbiology. CC BY 4.0. https://openstax.org/details/books/microbiology.
Figure 2.92: This patient has edema in the tissue of the right hand. Attributed to OpenStaxCNX. CC BY 3.0 Unported. https://commons.wikimedia.org/wiki/File:2707_Edema_of_Right_Hand_Due_to_Allergic_Reaction.jpg
Figure 2.93: Hyaluronan is a polymer found in the layers of epidermis that connect adjacent cells. (c) Rice University. OpenStax Microbiology. CC BY 4.0. https://openstax.org/details/books/microbiology.
Figure 2.94: Lipopolysaccharide is composed of lipid A, a core glycolipid, and an O-specific polysaccharide side chain. (c) Rice University. OpenStax Microbiology. CC BY 4.0. https://openstax.org/details/books/microbiology.
Figure 2.95: In A-B toxins, the B component binds to the host cell through its interaction with specific cell surface receptors. Modification of work by (c) “Biology Discussion Forum”/YouTube. CC BY 4.0.
Figure 2.96: The mechanism of the diphtheria toxin inhibiting protein synthesis. (c) Rice University. OpenStax Microbiology. CC BY 4.0. https://openstax.org/details/books/microbiology.
Figure 2.97: Mechanisms of botulinum and tetanus toxins. Left: modification of work by Centers for Disease Control and Prevention. Right: (c) Rice University. OpenStax Microbiology. CC BY 4.0. https://openstax.org/details/books/microbiology.
Figure 2.98: (a) A micrograph of capsules around bacterial cells. Left: modification of work by Centers for Disease Control and Prevention. Public domain. Middle and right: (c) Rice University. OpenStax Microbiology. CC BY 4.0. https://openstax.org/details/books/microbiology.
Figure 2.99: Antigenic drift and antigenic shift in influenza viruses. (c) Rice University. OpenStax Microbiology. CC BY 4.0. https://openstax.org/details/books/microbiology.
Text References
- R. Sinclair et al. “Persistence of Category A Select Agents in the Environment.” Applied and Environmental Microbiology 74 no. 3 (2008):555–563. ↵
- T.J. Silhavy, D. Kahne, S. Walker. “The Bacterial Cell Envelope.” Cold Spring Harbor Perspectives in Biology 2 no. 5 (2010):a000414. ↵
- L. Gana, S. Chena, G.J. Jensena. “Molecular Organization of Gram-Negative Peptidoglycan.” Proceedings of the National Academy of Sciences of the United States of America 105 no. 48 (2008):18953–18957 ↵
- J.A. Garnetta et al. “Structural Insights Into the Biogenesis and Biofilm Formation by the Escherichia coli Common Pilus.” Proceedings of the National Academy of Sciences of the United States of America 109 no. 10 (2012):3950–3955. ↵
- T. Proft, E.N. Baker. “Pili in Gram-Negative and Gram-Positive Bacteria—Structure, Assembly and Their Role in Disease.” Cellular and Molecular Life Sciences 66 (2009):613. ↵
- C.R. Woese. “Bacterial Evolution.” Microbiological Review 51 no. 2 (1987):221–271. ↵
- H. Reichenbach. “Myxobacteria, Producers of Novel Bioactive Substances.” Journal of Industrial Microbiology & Biotechnology 27 no. 3 (2001):149–156. ↵
- S. Suerbaum, P. Michetti. “Helicobacter pylori infection.” New England Journal of Medicine 347 no. 15 (2002):1175–1186. ↵
- R.C. Fuller et al. “Carbon Metabolism in Chromatium.” Journal of Biological Chemistry 236 (1961):2140–2149. ↵
- T.T. Selao et al. “Comparative Proteomic Studies in Rhodospirillum rubrum Grown Under Different Nitrogen Conditions.” Journal of Proteome Research 7 no. 8 (2008):3267–3275. ↵
- A. De los Rios et al. “Ultrastructural and Genetic Characteristics of Endolithic Cyanobacterial Biofilms Colonizing Antarctic Granite Rocks.” FEMS Microbiology Ecology 59 no. 2 (2007):386–395. ↵
- T. Cavalier-Smith. “Membrane Heredity and Early Chloroplast Evolution.” Trends in Plant Science 5 no. 4 (2000):174–182. ↵
- S. Zhang, D.A. Bryant. “The Tricarboxylic Acid Cycle in Cyanobacteria.” Science 334 no. 6062 (2011):1551–1553. ↵
- A. Cain et al. “Cyanobacteria as a Biosorbent for Mercuric Ion.” Bioresource Technology 99 no. 14 (2008):6578–6586. ↵
- C.S. Ku et al. “Edible Blue-Green Algae Reduce the Production of Pro-Inflammatory Cytokines by Inhibiting NF-κB Pathway in Macrophages and Splenocytes.” Biochimica et Biophysica Acta 1830 no. 4 (2013):2981–2988. ↵
- I. Stewart et al. "Cyanobacterial Poisoning in Livestock, Wild Mammals and Birds – an Overview." Advances in Experimental Medicine and Biology 619 (2008):613–637. ↵
- J. Flegr et al. “Toxoplasmosis—A Global Threat. Correlation of Latent Toxoplasmosis With Specific Disease Burden in a Set of 88 Countries.” PloS ONE 9 no. 3 (2014):e90203. ↵
- J. Flegr. “Effects of Toxoplasma on Human Behavior.” Schizophrenia Bull 33, no. 3 (2007):757–760. ↵
- Won K, Kruszon-Moran D, Schantz P, Jones J. “National seroprevalence and risk factors for zoonotic Toxocara spp. infection.” In: Abstracts of the 56th American Society of Tropical Medicine and Hygiene; Philadelphia, Pennsylvania; 2007 Nov 4-8. ↵
- N. Philippe et al. “Pandoraviruses: Amoeba Viruses with Genomes up to 2.5 Mb Reaching that of Parasitic Eukaryotes.” Science 341, no. 6143 (2013): 281–286. ↵
- J. Cohen. “What’s Old Is New: 1918 Virus Matches 2009 H1N1 Strain. Science 327, no. 5973 (2010): 1563–1564.National Institute of Neurological Disorders and Stroke. “Creutzfeldt-Jakob Disease Fact Sheet.” http://www.ninds.nih.gov/disorders/cjd/detail_cjd.htm (accessed December 31, 2015) / https://www.ninds.nih.gov/health-information/disorders/creutzfeldt-jakob-disease (accessed March 19, 2025). ↵
- M. Otto. “Staphylococcus epidermidis--The ‘Accidental’ Pathogen.” Nature Reviews Microbiology 7 no. 8 (2009):555–567. ↵
- D. Davies. “Understanding Biofilm Resistance to Antibacterial Agents.” Nature Reviews Drug Discovery 2 (2003):114–122. ↵
- V. Meka. “Panton-Valentine Leukocidin.” http://www.antimicrobe.org/h04c.files/history/PVL-S-aureus.asp (accessed before 2025) / https://web.archive.org/web/20220504110622/http://www.antimicrobe.org/h04c.files/history/PVL-S-aureus.asp (accessed March 26, 2025) ↵