1 Neuron and astrocyte metabolism
Learning Objectives
- Describe the metabolic pathway of glucose and its metabolites and how they are utilized in the brain, and list the major energy-consuming processes in the brain.
- Describe the role of glial cells in neuron metabolism.
- Evaluate the interaction between astrocytes and neurons in the regulation of cerebral blood flow.
- Compare the metabolic profile of neurons and astrocytes.
- Review basic metabolic pathways, including: glycolysis, glycogen synthesis/olysis, transaminations, and glutathione synthesis.
The adult brain consumes about 25 percent of the glucose-derived energy and 20 percent of oxygen is dedicated to cerebral functions. The primary fuel to support the high energy demands of this tissue is supplied in the form of glucose, however, not all neuronal tissues oxidize glucose to the same extent. This section will address the distinct metabolic differences between astrocyte and neuronal metabolic profiles and how this interplay is essential for brain metabolic homeostasis.
As a brief review, glucose is taken up by the brain in an insulin-independent manner. The brain oxidizes glucose under most conditions with the exception of starvation states. Once the glucose is phosphorylated to glucose 6-phosphate (by hexokinase), it has three potential fates (figure 1.1):
- Glycolysis (either leading to lactate production or mitochondrial metabolism),
- Pentose phosphate pathway (PPP), or
- Glycogen synthesis (only in astrocytes).
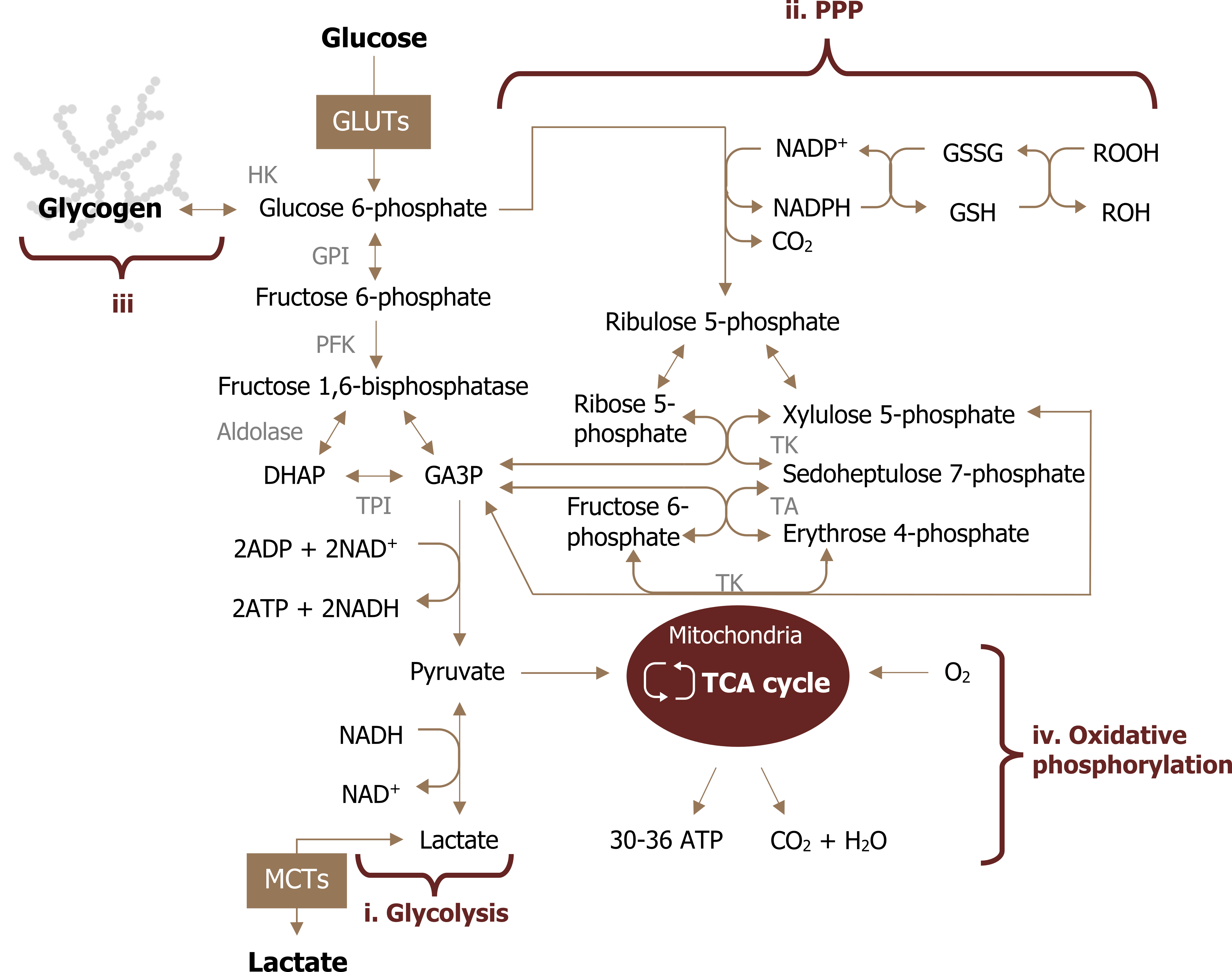
What is unique to the brain is that not all cell types oxidize glucose to the same extent, but there is a tight coupling that exists between cell types to support both energy demand and glutamate-mediated neurotransmission. This compartmentalized, coupled metabolism between neurons and astrocytes is essential for ATP production, neuronal excitability, and adaptations to stress.
Note
In the context of this chapter, oxidative metabolism refers to the oxidation of a substrate (glucose) through mitochondrial metabolism versus glycolytic metabolism, which refers to the oxidation of glucose to lactate.
Neuron metabolism
To support the high energy demands imposed on neurons, they sustain a high rate of oxidative metabolism for ATP production compared to astrocytes. Despite this high rate of mitochondrial metabolism, glucose uptake is reduced when compared to astrocytes, in part due to the use of lactate as an energy source. Neurons show a preference for lactate over glucose when both substrates are present. There are several reasons why sustaining a low glycolytic rate but high oxidative rate is preferred in this tissue:
- The bifunctional enzyme phosphofructokinase 2 (PFK2) is virtually absent in neurons, due to its constant proteasomal degradation. This enzyme is responsible for the generation of fructose 2,6-bisphosphate, which is an allosteric activator of the glycolytic enzyme phosphofructokinase 1 (PFK1).
- Elevated glycolytic flux impairs metabolism through the PPP. Neurons purposefully reduce glycolytic flux to maintain metabolism through the PPP—which is essential for the production of NADPH through the reaction catalyzed by glucose 6-phosphate dehydrogenase.
To accommodate these two processes, neurons preferentially utilize lactate, which can be readily converted to pyruvate and enter the mitochondria (figure 1.2). The lactate required for neuronal metabolism is produced by astrocytic glucose oxidation and is discussed below.
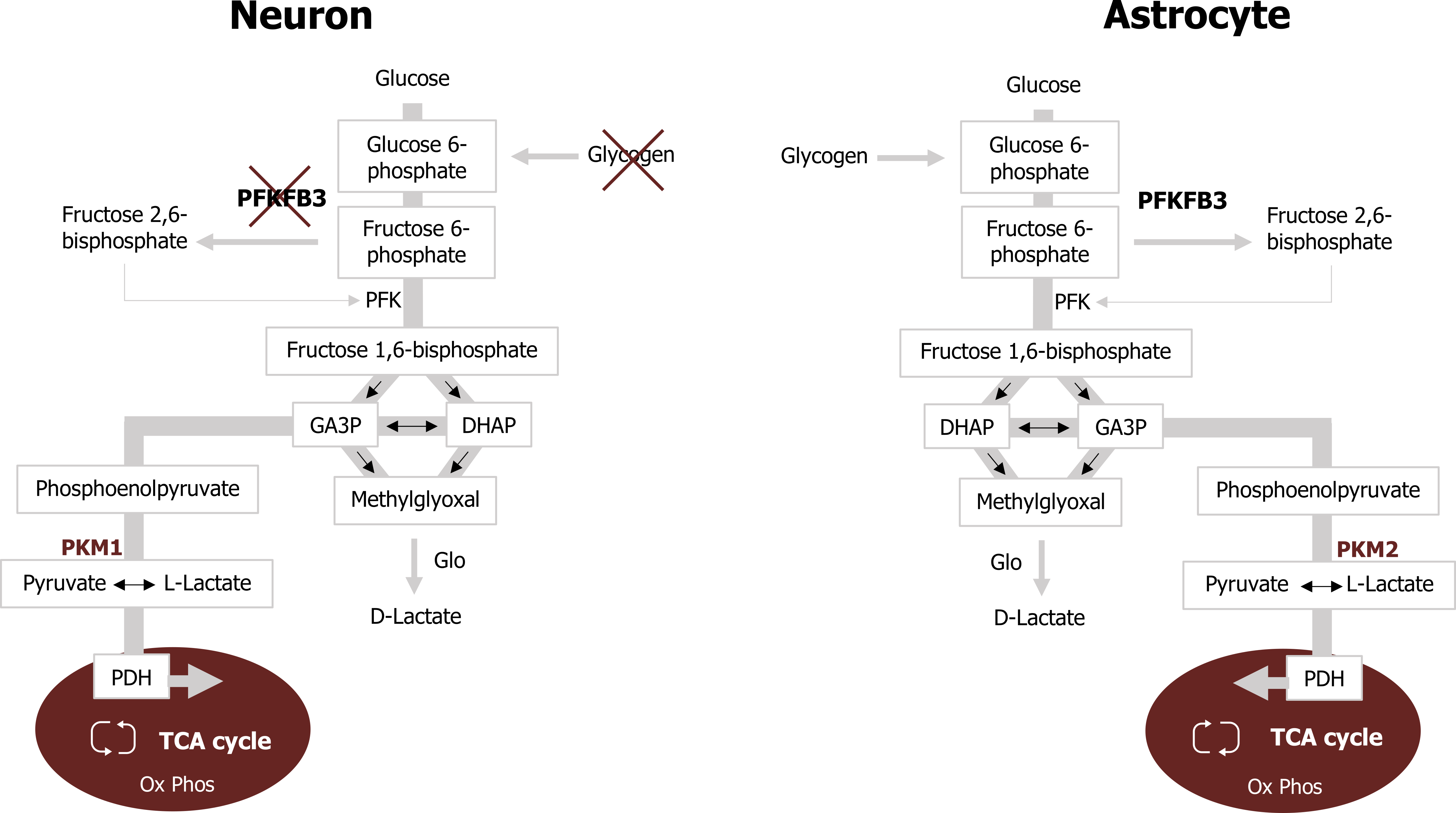
Astrocyte metabolism
Within the brain, astrocytes outnumber neurons but have reduced demand for ATP. Although astrocytes display lower oxidative rates when compared to neurons, they have elevated glycolytic rates and avidly take up glucose to support this. Astrocytes primarily metabolize glucose to lactate (rather than through mitochondrial metabolism) and release the lactate in the extracellular space. Astrocytes support this glycolytic profile through the alteration of several key glycolytic enzymes.
- Astrocytes have increased expression of PFK2, which assists with the elevated glycolytic rate via the allosteric activation of PFK1 by fructose 2,6-bisphosphate.
- Astrocytes have reduced expression of the aspartate/glutamate carrier (AGC), a component of the malate-aspartate shuttle, which facilitates the transfer of reducing equivalents from the cytosol to the mitochondria. As a result, the conversion of pyruvate to lactate in the cytosol is needed to maintain a high NAD+/NADH ratio, which is essential to sustain a high glycolytic rate.
- Finally, the conversion of pyruvate to lactate in astrocytes is also favored by both reduced expression and phosphorylation-mediated inactivation of the pyruvate dehydrogenase complex (figure 1.2).
Astrocyte-mediated neurotransmitter recycling
To terminate synaptic transmission and maintain neuronal excitability, astrocytes play a key role in the rapid removal of neurotransmitters from the synaptic cleft. The removal of glutamate is specifically critical as this is the primary excitatory neurotransmitter, and overstimulation of glutamate receptors is highly toxic to neurons.
Astrocytes recycle glutamate through sodium-dependent high-affinity glutamate transporters. Following glutamate uptake, astrocytes also play an important role in transferring this neurotransmitter back to neurons. This transfer is achieved by a process called the glutamate-glutamine cycle, which involves both glutamine synthetase (GS) and glutaminase (GLS) (figure 1.3).
- First, glutamate is converted to glutamine by the astrocyte-specific enzyme GS.
- Glutamine is then transferred to neurons and converted back to glutamate via deamination by GLS.
It is also important to note that astrocytes are the only neural cell type expressing pyruvate carboxylase, a key enzyme in the main anaplerotic pathway in the brain. This allows astrocytes to effectively synthesize glutamate from glucose, making them the cell type responsible for the replenishment of brain glutamate.
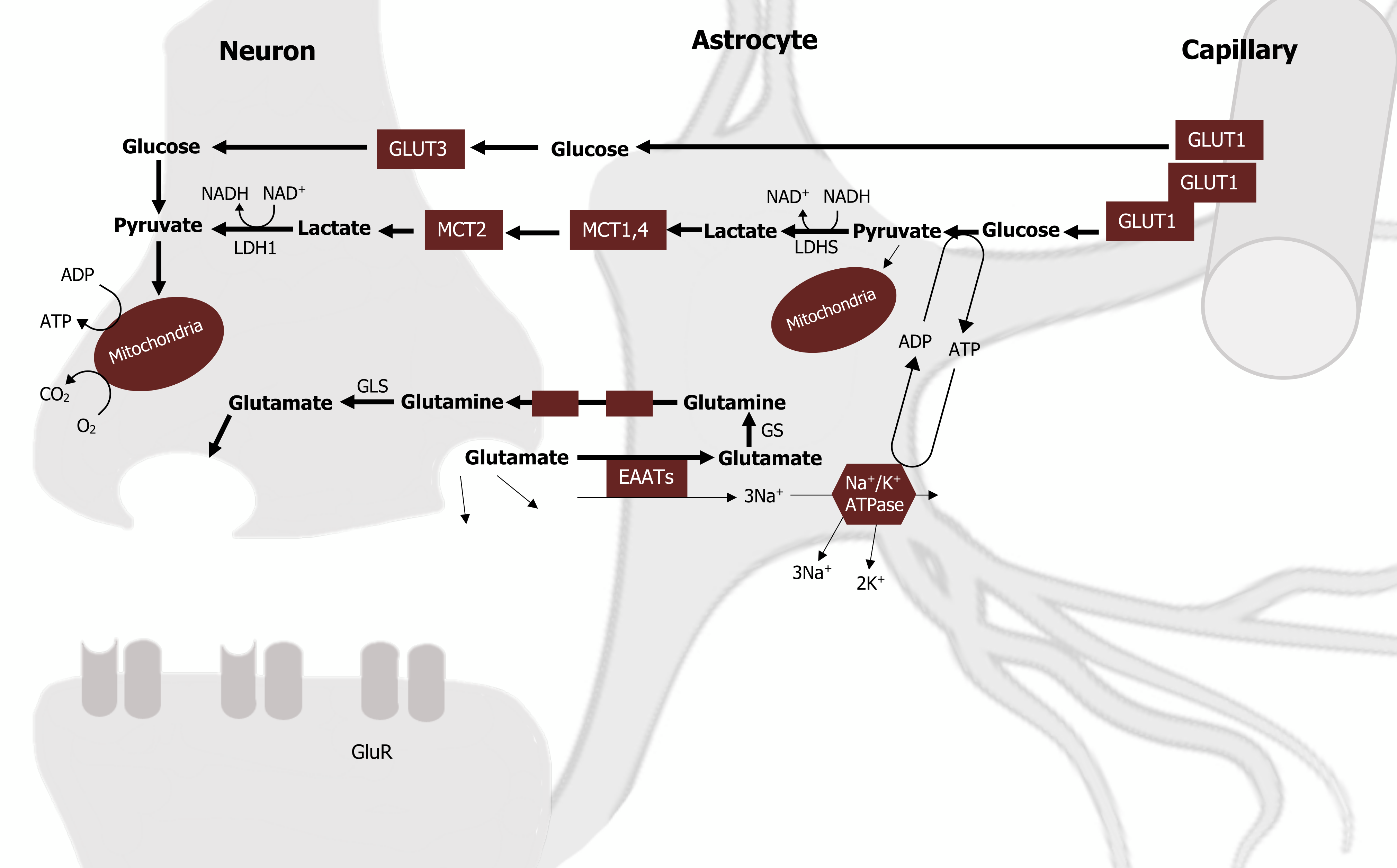
Astrocyte-neuron lactate shuttle
To this point, we have addressed the need for lactate in the neuron to sustain high demands for ATP, astrocyte glycolytic activity and elevated glucose uptake, and the role of the astrocyte in glutamate recycling. These three processes are not unrelated, and the juxtaposition in energy needs and glucose uptake between these two cell types can be reconciled when we address the role of the astrocyte-neuron lactate shuttle (ANLS).
The premise of this shuttle is threefold:
- Neuronal activity increases extracellular glutamate (via glutamatergic neurotransmission), which is avidly taken up via an Na+-dependent mechanism.
- The resulting increase in intracellular Na+ activates the Na+/K+ ATP-ase and increases ATP consumption.
- The fall of ATP increases lactate production, which is released into the extracellular space.
Finally, lactate can be used as an energy substrate for neurons for oxidative-derived ATP production (figure 1.3).
Astrocyte glycogen stores
Glycogen is the largest energy reserve of the brain and provides a source of glucose that can be rapidly mobilized without ATP. Stores of glycogen in the brain are almost exclusively localized to astrocytes. Again, this observation raises the question as to why the main energy reserve in the brain is found in astrocytes rather than the high-energy-requiring neurons.
Much like the lactate shuttle, glycogen metabolism relies heavily on the interaction between the two cell types to preserve metabolic homeostasis within the brain environment. Glycogen mobilization can support astrocyte metabolism, but it also increases lactate production and the concentration of lactate in the extracellular space. Considering the role of astrocytic glucose-derived lactate in fueling neuronal energy needs, this coupling of these compartmentalized metabolic processes helps preserve the function of each cell type.
Glycogen also has other roles in the normal brain. The amount of glycogen is under dynamic control of neurotransmitters. For example, decreased neuronal activity, such as in sleep, correlates with increased levels of brain glycogen, while an increase in neuronal activity, such as in the awake brain, is associated with a decrease in glycogen levels in active areas.
As a whole, these observations demonstrate that astrocytic glycogen plays an important and active role in complex brain physiological functions, in particular through an astrocyte-to-neuron transfer of energy metabolites in the form of lactate.
The role of astrocyte-neuron interactions to reduce oxidative stress
Oxidative injury is a key feature of several neuropathological conditions such as stroke, traumatic brain injury, and neurodegenerative diseases. There are several factors that contribute to increased brain vulnerability to oxidative stress, including its high rate of oxidative energy metabolism (a process inevitably generating reactive oxygen species (ROS) as a byproduct), its high unsaturated fatty acids content (which are prone to lipid peroxidation), and its relatively low intrinsic antioxidant capacity.
Although neurons have a higher proportion of oxidative metabolism, they have few defenses against oxidative stress. Astrocytes, alternatively, have significant levels of ROS-detoxifying enzymes, including glutathione, heme oxygenase 1, glutathione peroxidase, glutathione S-transferase, catalase, and thioredoxin reductase. As a result, astrocytes are much more resistant to cellular damage by H2O2, NO, peroxinitrites, and 6-hydroxydopamine compared to neurons.
Astrocytes also play an important protective role for neurons, suggesting that neurons are dependent on the high antioxidant potential of astrocytes for their own defense against oxidative stress.
One way in which astrocytes provide protection is through the shuttling of glutathione (GSH) precursors from astrocytes to neurons. GSH—the most abundant antioxidant molecule in the brain—either acts directly as a ROS scavenger or can be used as a substrate for glutathione S-transferase or glutathione peroxidase. Both cell types can synthesize the GSH tripeptide, but neurons are highly dependent on astrocytes for the supply of the precursor amino acids necessary for their own GSH synthesis.
The reduction of peroxides catalyzed by glutathione peroxidase generates glutathione disulfide (GSSG, the oxidized form of GSH). GSH can subsequently be regenerated from GSSG by the action of the enzyme glutathione reductase, using NADPH as an electron donor. This process is essential for the maintenance of GSH in its reduced form, and thus for its availability for the detoxification of ROS. As a consequence, constant NADPH supply is essential for the maintenance of the cellular redox state.
NADPH production is mainly achieved by the metabolism of glucose via the PPP, thereby coupling glutathione recycling to glucose utilization. NADPH is more abundant in astrocytes than in neurons, and astrocytes have a higher basal PPP activity rate and a better capacity to stimulate this pathway in response to oxidative stress.
Astrocyte-neuron impact on cerebral blood flow
The metabolic interplay between astrocytes and neurons goes beyond substrate usage and availability to impact cerebral blood flow through both vasoconstriction and vasodilation. Interestingly, these opposite effects on the vascular tone involve different but parallel signaling cascades in astrocytes.
Briefly, neuronal release of glutamate can stimulate the metabotropic glutamate receptors (mGluR) on astrocytes. This in turn activates phospholipase C and increases intracellular calcium. Ca2+ transients in astrocytes activate cytosolic phospholipase A2 (PLA2), thus producing arachidonic acid (AA). AA can be used for the synthesis of prostaglandins, which can have different types of vasodilating effects. In parallel, AA can diffuse to arteriolar smooth muscle cells, where it is converted to 20-hydroxyeicosatetraenoic acid (20-HETE), which has a vasoconstricting effect. Whether the predominant impact on vascular tone is dilation or constriction depends on levels of lactate and adenosine in the extracellular space.
During brain activation, consistent with low O2, an increase in lactate in the extracellular space will enhance vasodilation by assisting in the accumulation of prostaglandin E2 (PGE2). Elevated adenosine also enhances vasodilation but through an inhibitory effect on the synthesis of 20-HETE. This is an interesting observation and suggests that cerebral blood flow is largely controlled by glycolytic (vs. oxidative) metabolism and that increased cerebral blood flow correlates with elevations in lactate concentration (rather than O2 consumption).
References and resources
Text
Bélanger, M., I. Allaman, and P. J. Magistretti. “Brain Energy Metabolism: Focus on Astrocyte–Neuron Metabolic Cooperation.” Cell Metabolism 14, no. 6 (December 2011): 724–738, https://doi.org/10.1016/j.cmet.2011.08.016.
Le, T., and V. Bhushan. First Aid for the USMLE Step 1, 29th ed. New York: McGraw Hill Education, 2018, 87, 314–315.
Lieberman, M., and A. Peet, eds. Marks’ Basic Medical Biochemistry: A Clinical Approach, 5th ed. Philadelphia: Wolters Kluwer Health/Lippincott Williams & Wilkins, 2018, Chapter 46: Metabolism of the Nervous System.
Magistretti, P. J., and I. Allaman. “A Cellular Perspective on Brain Energy Metabolism and Functional Imaging.” Neuron 86, no. 4 (May 2015): 883–901, https://doi.org/10.1016/j.neuron.2015.03.035.
Figures
Figure 1.1: Potential fates of glucose oxidation. Magistretti, P. J., and I. Allaman. “A Cellular Perspective on Brain Energy Metabolism and Functional Imaging.” Neuron 86, no. 4 (May 2015): 883–901, https://doi.org/10.1016/j.neuron.2015.03.035. Adapted under fair use.
Figure 1.2: Comparison of neuron andd astrocyte metabollism. Magistretti, P. J., and I. Allaman. “A Cellular Perspective on Brain Energy Metabolism and Functional Imaging.” Neuron 86, no. 4 (May 2015): 883–901, https://doi.org/10.1016/j.neuron.2015.03.035. Adapted under fair use.
Figure 1.3: Lactate and glutamate shuttling between the astrocyte and neuron. Magistretti, P. J., and I. Allaman. “A Cellular Perspective on Brain Energy Metabolism and Functional Imaging.” Neuron 86, no. 4 (May 2015): 883–901, https://doi.org/10.1016/j.neuron.2015.03.035. Adapted under fair use. Added diagram of an astrocyte by Cancer Research UK, CC BY 4.0., Wikimedia Commons, https://commons.wikimedia.org/wiki/F…l_CRUK_029.svg.