17 Origin of the Universe and Our Solar System
Learning Objectives
By the end of this chapter, students should be able to:
- Explain the formation of the universe and how we observe it.
- Understand the origin of our Solar System.
- Describe how the objects in our Solar System are identified, explored, and characterized.
- Describe the types of bodies in our Solar System, their locations, and how they formed.
- Explain what influences the temperature of a planet’s surface.
- Describe different methods for dating planets and the age of the Solar System.
- Discuss the assumption underlying the Copernican principle and outline its implications for modern-day astronomers.
- Identify where in the Solar System life is most likely sustainable and why.
- Understand the questions underlying the Fermi paradox.
The universe began 13.77 billion years ago when energy, matter, and space expanded from a single point. Evidence for the big bang is the cosmic “afterglow” from when the universe was still very dense, and red-shifted light from distant galaxies, which tell us the universe is still expanding.
The big bang produced hydrogen, helium, and lithium, but heavier elements come from nuclear fusion reactions in stars. Large stars make elements such as silicon, iron, and magnesium, which are important in forming terrestrial planets. Large stars explode as supernovae and scatter the elements into space.
Planetary systems begin with the collapse of a cloud of gas and dust. Material drawn to the center forms a star, and the remainder forms a disk around the star. Material within the disk clumps together to form planets. In our Solar System, rocky planets are closer to the Sun, and ice and gas giants are farther away. This is because temperatures near the Sun were too high for ice to form, but silicate minerals and metals could solidify.
Early Earth was heated by radioactive decay, collisions with bodies from space, and gravitational compression. Heating melted Earth, causing molten metal to sink to Earth’s center and form a core, and causing silicate melt to float to the surface and form the mantle and crust. A collision with a planet the size of Mars knocked debris into orbit around Earth, and the debris coalesced into the Moon. Earth’s atmosphere is the result of volcanic degassing, contributions by comets and meteorites, and photosynthesis.
The search for exoplanets has identified many Earth-sized rocky exoplanets, some of which are in the habitable zones of their stars. These are thought to be rocky worlds like Earth, but the compositions of these planets are not known for certain.
17.1 The Big Bang
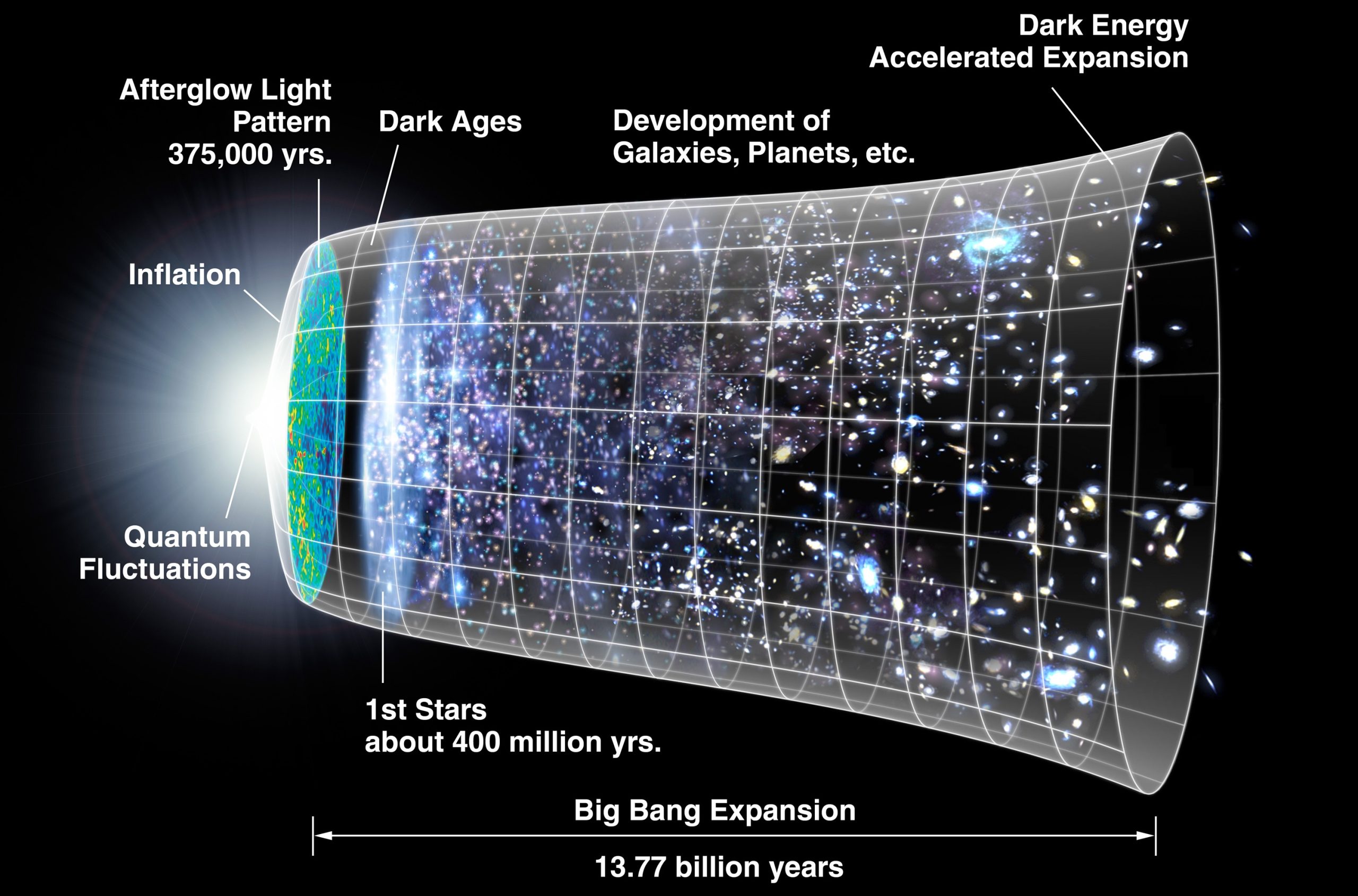
According to the big bang theory, the universe blinked violently into existence 13.77 billion years ago. The big bang is often described as an explosion, but imagining it as an enormous fireball isn’t accurate. The big bang involved a sudden expansion of matter, energy, and space from a single point. The kind of Hollywood explosion that might come to mind involves expansion of matter and energy within space, but during the big bang, space itself was created.
At the start of the big bang, the universe was too hot and dense to be anything but a sizzle of particles smaller than atoms, but as it expanded, it also cooled. Eventually some of the particles collided and stuck together. Those collisions produced the simplest elements, hydrogen and helium, which are also the most common elements in the universe, along with a small amount of lithium. Today, hydrogen is still the most abundant element in the universe with its simple structure of just one proton and one electron.
You may wonder how a universe can be created out of nothing or how we can know that the big bang happened at all. Creating a universe out of nothing is mostly beyond the scope of this chapter, but there is a way to think about it. The particles that make up the universe have opposites that cancel each other out, similar to the way that we can add the numbers 1 and −1 to get zero (also known as “nothing”). As far as the math goes, having zero is exactly the same as having a 1 and a −1. It is also exactly the same as having a 2 and a −2, a 3 and a −3, two −1s and a 2, and so on. In other words, nothing is really the potential for something if you divide it into its opposite parts. As for how we can know that the big bang happened at all, there are very good reasons to accept that it is indeed how our universe came to be.
17.1.1 Looking Back to the Early Stages of the Big Bang
The notion of seeing the past is often used metaphorically when we talk about ancient events, but in this case, it is meant literally. In our everyday experience, when we watch an event take place, we perceive that we are watching it as it unfolds in real time. In fact, this isn’t true. To see the event, light from that event must travel to our eyes. Light travels very rapidly, but it does not travel instantly. If we were watching a digital clock one meter away from us change from 11:59 a.m. to 12:00 p.m., we would actually see it turn to 12:00 p.m. three billionths of a second after it happened. This isn’t enough of a delay to cause us to be late for an appointment, but the universe is a very big place, and the “digital clock” in question is often much, much farther away. In fact, the universe is so big that it is convenient to describe distances in terms of light years, or the distance light travels in one year. What this means is that light from distant objects takes so long to get to us that we see those objects as they were at some considerable time in the past. For example, the star Proxima Centauri is 4.24 light years from the sun. If you viewed Proxima Centauri from Earth on January 1, 2015, you would actually see it as it appeared in early October 2010.
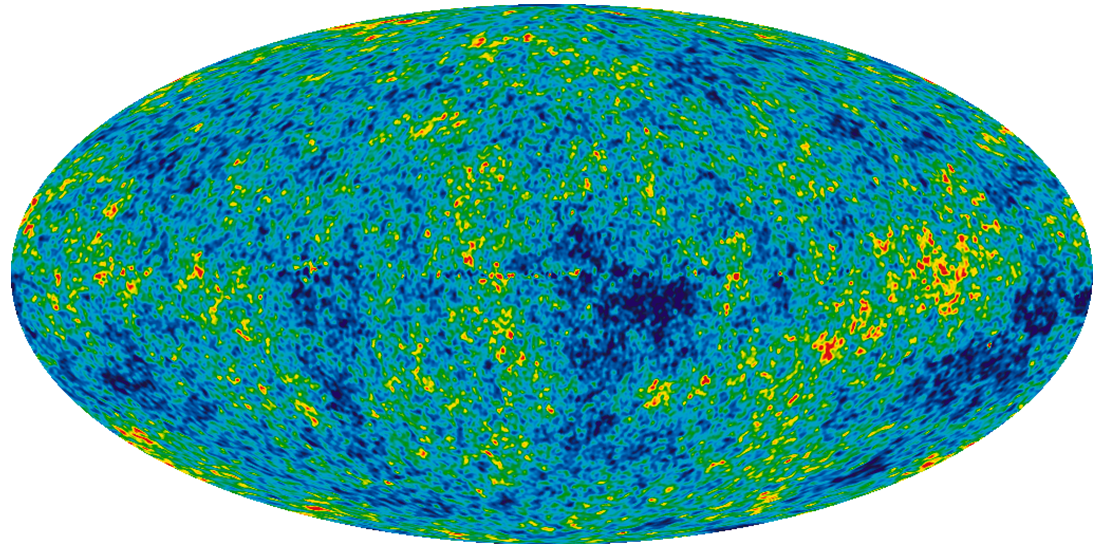
We now have tools that are powerful enough to look deep into space and see the arrival of light from early in the universe’s history. Astronomers can detect light from approximately 375,000 years after the big bang is thought to have occurred. Physicists tell us that, if the big bang happened, then particles within the universe would still be very close together at this time. They would be so close that light wouldn’t be able to travel far without bumping into another particle and getting scattered in another direction. The effect would fill the sky with glowing fog, the “afterglow” from the formation of the universe.
In fact, this is exactly what we see when we look at light from 375,000 years after the big bang. The fog is referred to as the cosmic microwave background (CMB), and it has been carefully mapped throughout the sky. The map displays the cosmic microwave background as temperature variations, but these variations translate to differences in the density of matter in the early universe. The red patches are the highest density regions, and the blue patches are the lowest density. Higher-density regions represent the eventual beginnings of stars and planets. The map has been likened to a baby picture of the universe.
17.1.2 The Big Bang is Still Happening, and We Can See the Universe Expanding

The expansion that started with the big bang never stopped. It continues today, and through observation, we can see large clusters of billions of stars (galaxies) moving away from us. Though the only exception is the Andromeda galaxy, with which we are on a collision course. The astronomer Edwin Hubble came to this conclusion when he observed that the light from other galaxies was red-shifted. The red shift is a consequence of the Doppler effect. This refers to how we see waves when the object that is creating the waves is moving toward us or away from us.
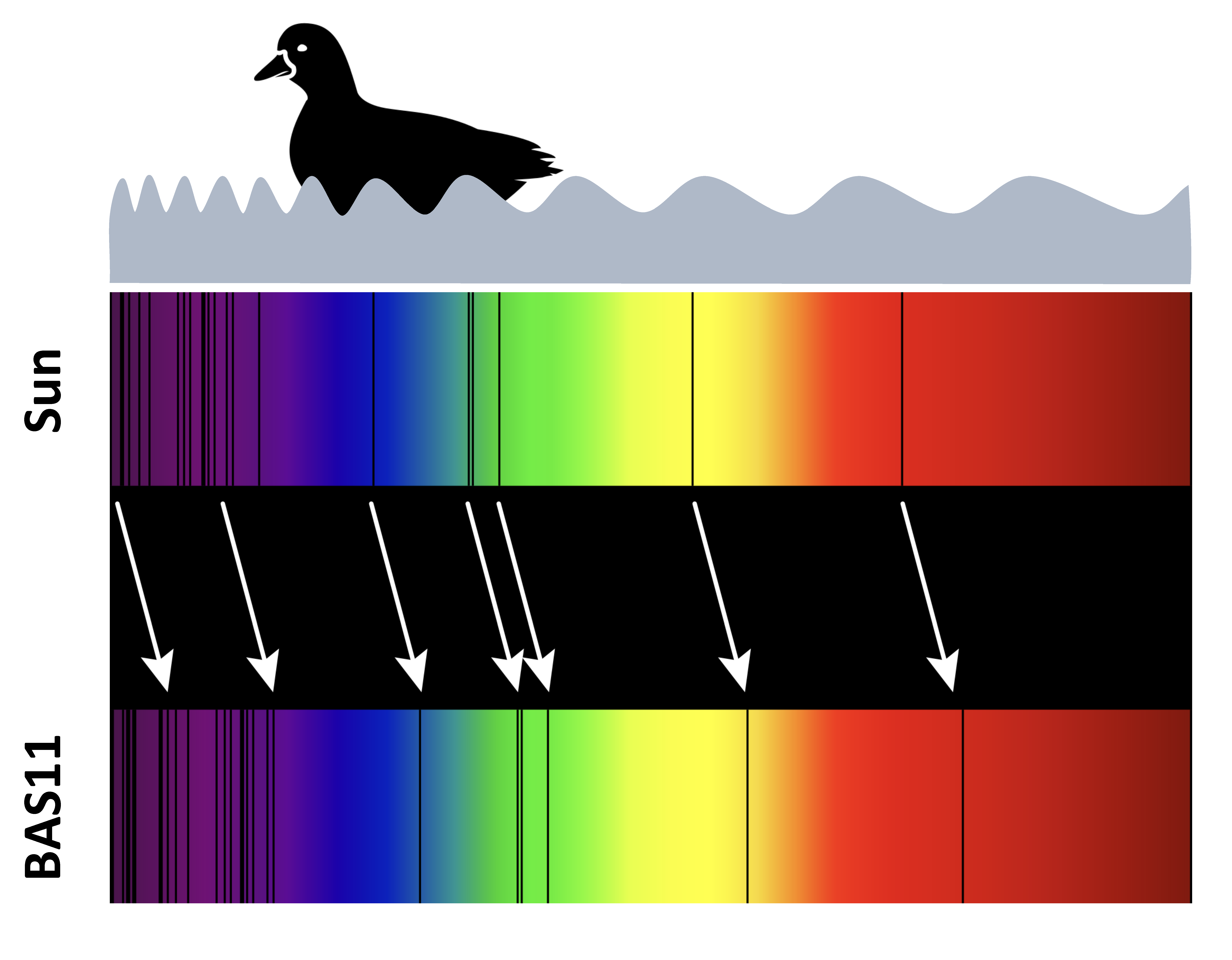
Before we get to the Doppler effect as it pertains to the red shift, let’s see how it works on something more tangible. The swimming duckling is generating waves as it moves through the water. It is generating waves that move forward as well as back, but notice that the ripples ahead of the duckling are closer to each other than the ripples behind the duckling. The distance from one ripple to the next is called the wavelength. The wavelength is shorter in the direction that the duckling is moving and longer as the duckling moves away.
As it pertains to air traveling in sound waves, the different wavelengths manifest as sounds with different pitches—the short wavelengths have a higher pitch, and the long wavelengths have a lower pitch. This is why the pitch of a car’s engine changes as the car races past you.
Take this quiz to check your comprehension of this section.
If you are using an offline version of this text, access the quiz for Section 17.1 via the QR code.
17.2 Overview of Our Planetary System
The Solar System consists of the Sun and many smaller objects: the planets, their moons and rings, and such “debris” as asteroids, comets, and dust. Decades of observation and spacecraft exploration have revealed that most of these objects formed together with the Sun about 4.5 billion years ago. They represent clumps of material that condensed from an enormous cloud of gas and dust. The central part of this cloud became the Sun, and a small fraction of the material in the outer parts eventually formed the other objects.
During the past 50 years, we have learned more about the Solar System than anyone imagined before the Space Age. In addition to gathering information with powerful new telescopes, we have sent spacecraft directly to many members of the planetary system. Planetary astronomy is the only branch of our science in which we can, at least vicariously, travel to the objects we want to study. With evocative names such as Voyager, Pioneer, Curiosity, and Pathfinder, our robot explorers have flown past, orbited, or landed on every planet, returning images and data that have dazzled both astronomers and the public. In the process, we have also investigated two dwarf planets, hundreds of fascinating moons, four ring systems, a dozen asteroids, and several comets (smaller members of our Solar System that we will discuss later).
Our probes have penetrated the atmospheres of Jupiter and Saturn and landed on the surfaces of Venus, Mars, our Moon, Saturn’s moon Titan, the asteroids Eros, Itokawa, Ryugu, and Bennu, and the comet Churyumov-Gerasimenko (usually referred to as 67P). Humans have set foot on the Moon and returned samples of its surface soil for laboratory analysis. We have flown a helicopter drone on Mars. We have even discovered other places in our Solar System that might be able to support some kind of life.

17.2.1 An Inventory
The Sun, a star that is brighter than about 80% of the stars in the galaxy, is by far the most massive member of the Solar System. It is an enormous ball about 1.4 million kilometers in diameter, with surface layers of incandescent gas and an interior temperature of millions of degrees.
Object | Percentage of total mass of Solar System |
---|---|
Sun | 99.8% |
Jupiter | 0.1% |
Comets | 0.0005–0.03% (estimate) |
All other planets and dwarf planets | 0.04% |
Moons and rings | 0.00005% |
Asteroids | 0.000002% (estimate) |
Cosmic dust | 0.0000001% (estimate) |
Table 17.1: Mass of members of the Solar System. Note that the Sun is by far the most massive member of the Solar System.
Most of the material of the planets in the Solar System is actually concentrated in the largest one, Jupiter, which is more massive than all the rest of the planets combined. Astronomers were able to determine the masses of the planets centuries ago using Kepler’s laws of planetary motion and Newton’s law of gravity to measure the planets’ gravitational effects on one another or on moons that orbit them. Today, we make even more precise measurements of their masses by tracking their gravitational effects on the motion of spacecraft that pass near them.
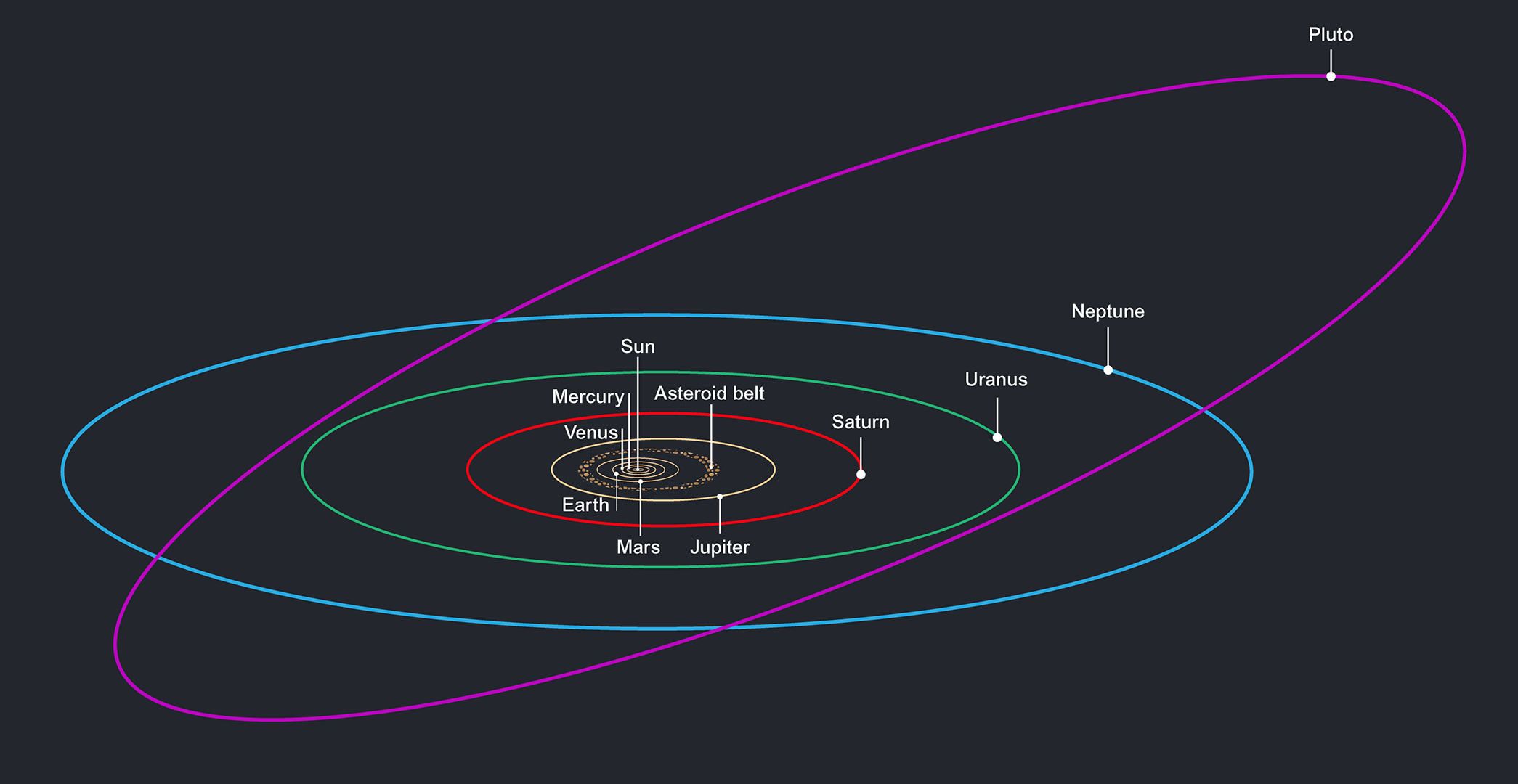
Beside Earth, five other planets were known to the ancients—Mercury, Venus, Mars, Jupiter, and Saturn—and two were discovered after the invention of the telescope: Uranus and Neptune. The eight planets all revolve in the same direction around the Sun. They orbit in approximately the same plane, like cars traveling on concentric tracks on a giant, flat racecourse. Each planet stays in its own “traffic lane,” following a nearly circular orbit about the Sun and obeying the “traffic” laws discovered by Galileo, Kepler, and Newton. Besides these planets, we have also been discovering smaller worlds beyond Neptune that are called trans-Neptunian objects or TNOs. The first to be found, in 1930, was Pluto, but others have been discovered during the twenty-first century. One of them, Eris, is about the same size as Pluto and has at least one moon (Pluto has five known moons). The largest TNOs are also classed as dwarf planets, as is the largest asteroid, Ceres. To date, more than 2,600 of these TNOs have been discovered, and one, called Arrokoth, was explored by the New Horizons spacecraft.
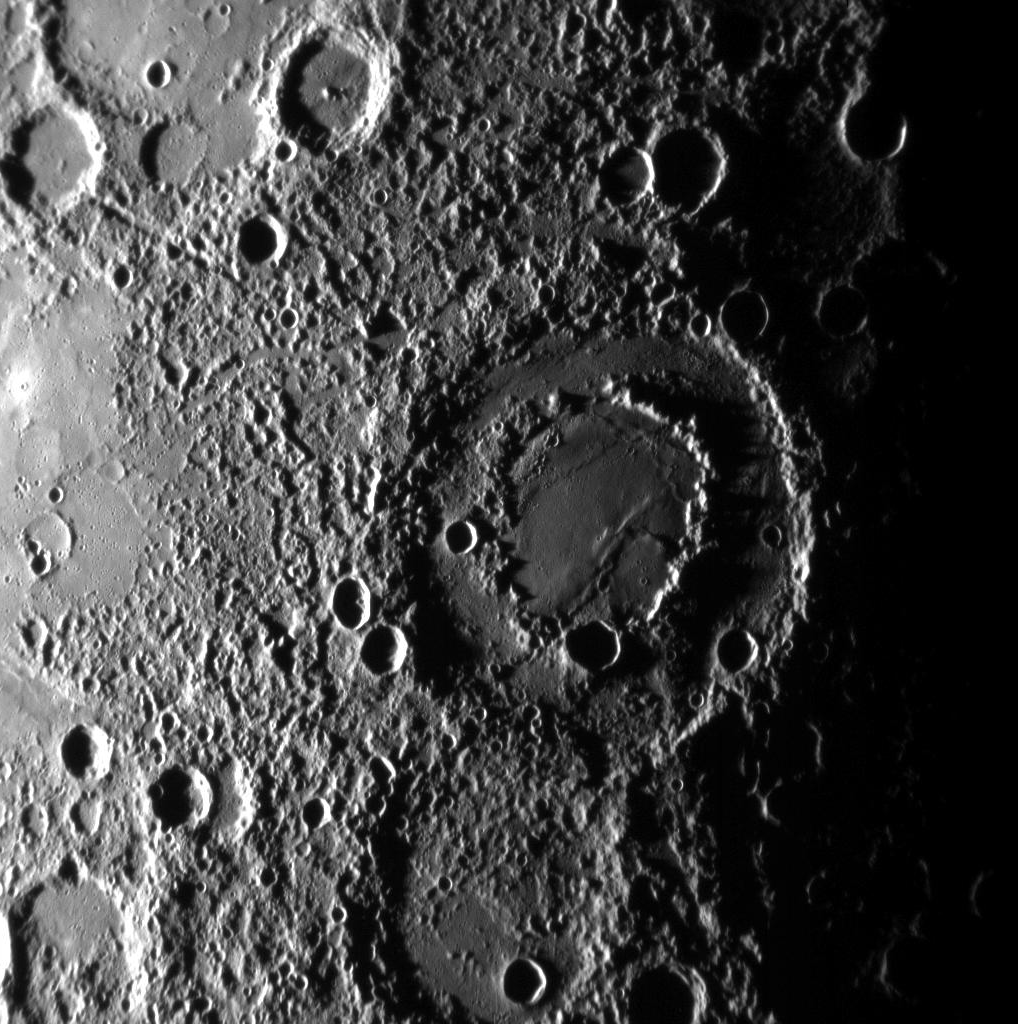
Each of the planets and dwarf planets also rotates (spins) about an axis running through it, and in most cases, the direction of rotation is the same as the direction of revolution about the Sun. The exceptions are Venus, which rotates backward (that is, in a retrograde direction), and Uranus and Pluto, which also have strange rotations, each spinning about an axis tipped nearly on its side. We do not yet know the spin orientations of the dwarf planets Eris, Haumea, and Makemake.
The rotation rates of the eight planets have been measured as follows. Mercury has a day that lasts 1,408 Earth hours, while Venus has the longest day of any planet at 5,832 Earth hours. Earth and Mars have similar day lengths, with Earth taking 24 hours to complete one rotation and Mars taking 25 hours. The gas giant rotate much faster than the terrestrial planets, with Jupiter having the shortest day at just 10 Earth hours for one rotation. Saturn is slightly slower, with a day length of 11 Earth hours. The two outermost planets, Uranus and Neptune, have day lengths of 17 Earth hours and 16 Earth hours, respectively.
The four planets closest to the Sun (Mercury through Mars) are called the inner, or terrestrial, planets. Often, the Moon is also discussed as a part of this group, bringing the total of terrestrial objects to five (we generally call Earth’s satellite “the Moon,” with a capital M, and the other satellites “moons,” with lowercase m’s). The terrestrial planets are relatively small worlds, composed primarily of rock and metal. All of them have solid surfaces that bear the records of their geological histories in the forms of craters, mountains, canyons, and volcanoes.
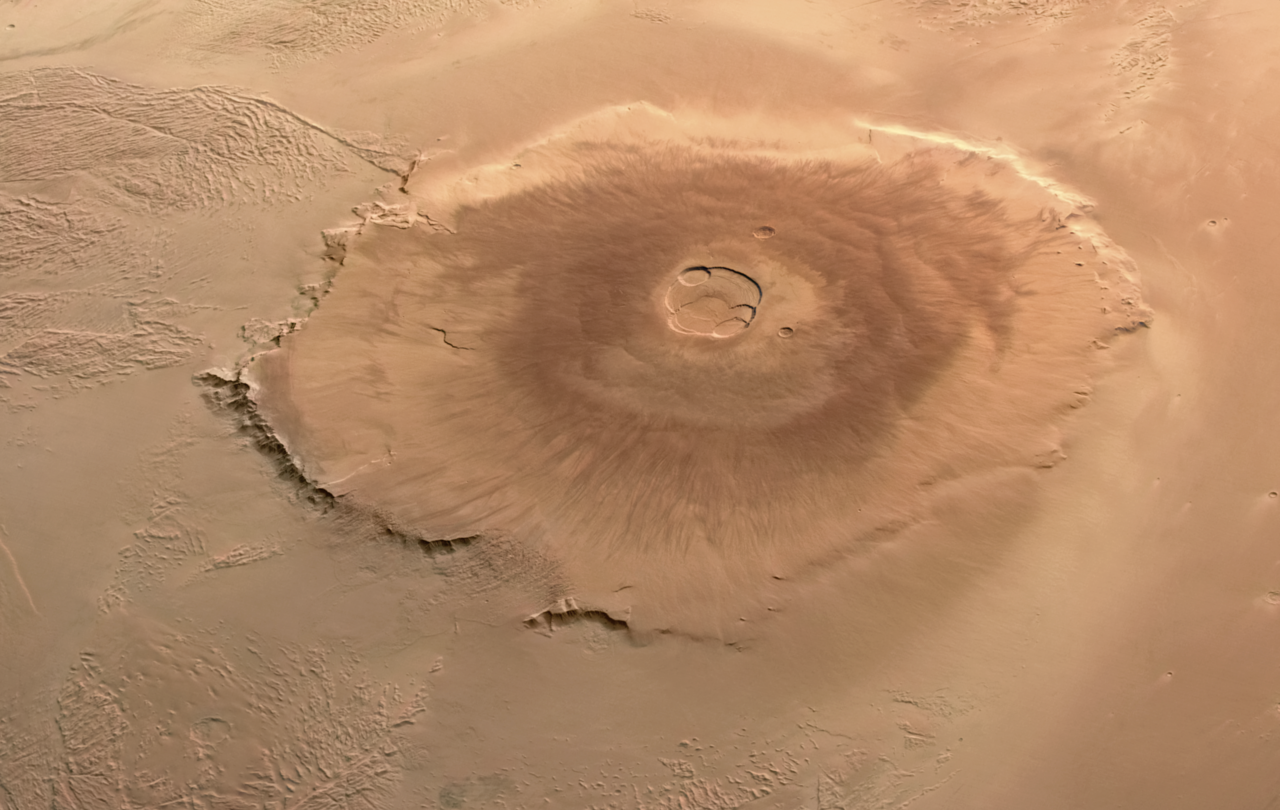
Although the terrestrial planets share similar geological features, the size and abundance of these features vary on each planet. For instance, volcanic rock is present on all four inner planets, but Venus has the most volcanoes, with over 1,600 major ones and many smaller ones. In contrast, Mars has fewer than 20 named volcanoes, but it boasts the largest volcanoes in the Solar System, including Olympus Mons, the tallest of them all.
Mars is also home to the largest canyon in the Solar System, the Valles Marineris, which stretches over 3,000 km in length, spans up to 600 km in width, and reaches depths of up to 8 km. By comparison, the Earth’s Grand Canyon in Arizona is 800 km long, 30 km across, and 1.8 km deep.
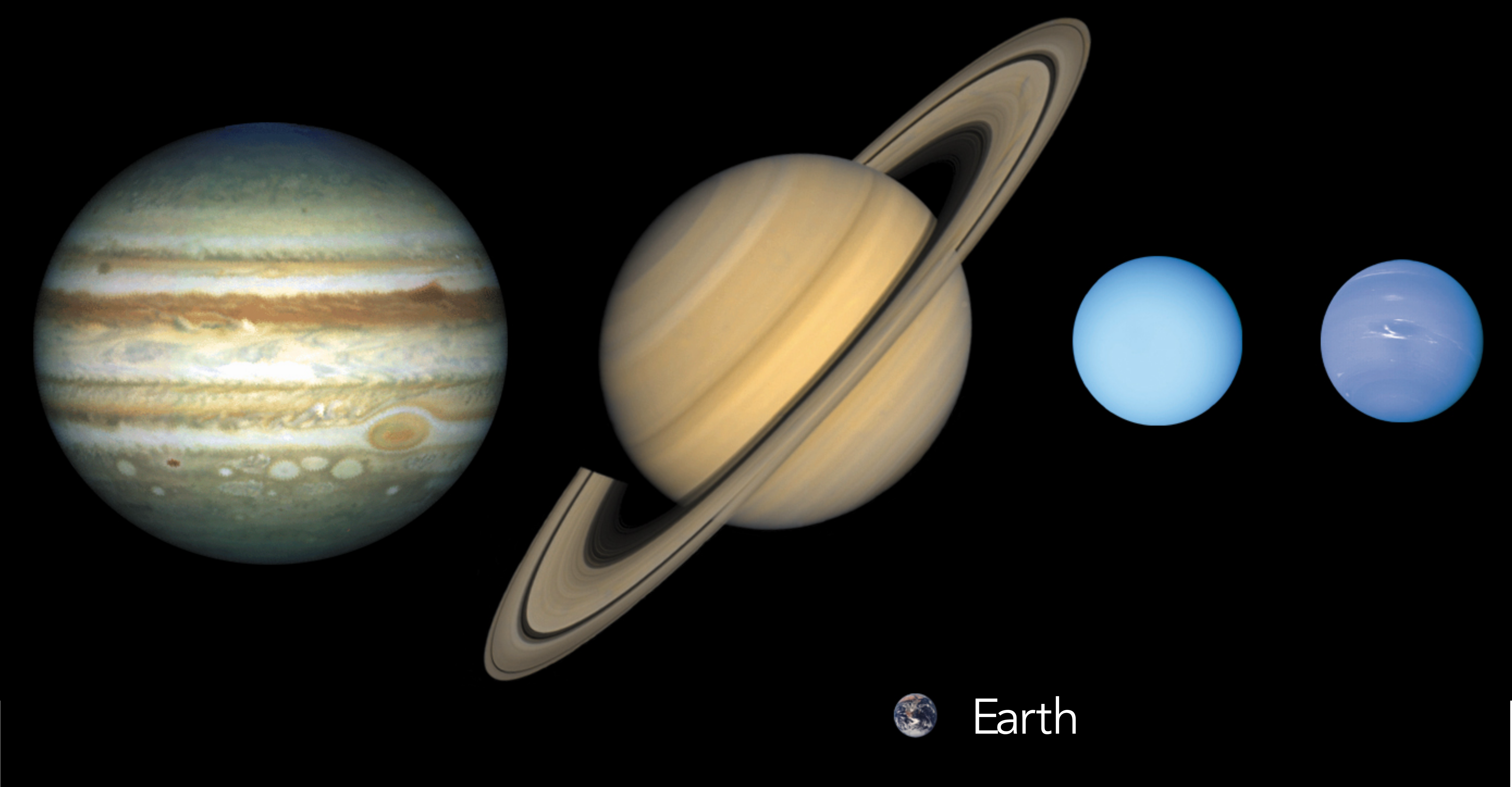
The next four planets (Jupiter through Neptune) are much larger and are composed primarily of lighter ices, liquids, and gases. We call these four the Jovian planets (after “Jove,” another name for Jupiter in mythology) or giant planets—a name they richly deserve. About 1,300 Earths could fit inside Jupiter, for example. These planets do not have solid surfaces on which future explorers might land. They are more like vast, spherical oceans with much smaller, dense cores.
Name | Distance from Sun (AU) | Revolution period (y) | Diameter (km) | Mass (1023 kg) | Density (g/cm3) |
---|---|---|---|---|---|
Mercury | 0.39 | 0.24 | 4,878 | 3.3 | 5.4 |
Venus | 0.72 | 0.62 | 12,120 | 48.7 | 5.2 |
Earth | 1.00 | 1.00 | 12,756 | 59.8 | 5.5 |
Mars | 1.52 | 1.88 | 6,787 | 6.4 | 3.9 |
Jupiter | 5.20 | 11.86 | 142,984 | 18,991 | 1.3 |
Saturn | 9.54 | 29.46 | 120,536 | 5,686 | 0.7 |
Uranus | 19.18 | 84.07 | 51,118 | 866 | 1.3 |
Neptune | 30.06 | 164.82 | 49,660 | 1,030 | 1.6 |

Near the outer edge of the system lies Pluto, which was the first of the distant icy worlds to be discovered beyond Neptune. Pluto was visited by a spacecraft, the NASA New Horizons mission, in 2015.
The outermost part of the Solar System is known as the Kuiper Belt, which is a scattering of rocky and icy bodies. Beyond that is the Oort cloud, a zone filled with small and dispersed ice traces. These two locations are where most comets form and continue to orbit, and objects found here have relatively irregular orbits compared to the rest of the Solar System. Pluto, formerly the ninth planet, is located in this region of space. The XXVI General Assembly of the International Astronomical Union (IAU) stripped Pluto of planetary status in 2006 because scientists discovered an object more massive than Pluto, which they named Eris. The IAU decided against including Eris as a planet and therefore excluded Pluto as well.
The IAU narrowed the definition of a planet to three criteria: (1) it must orbit a star (in our cosmic neighborhood, the Sun), (2) it must be big enough to have enough gravity to force it into a spherical shape, and (3) it must be big enough that its gravity cleared away any other objects of a similar size near its orbit around the Sun. Pluto passed the first two parts of the definition but not the third. Pluto and Eris are currently classified as dwarf planets.
17.2.2 Smaller Members of the Solar System
Most of the planets are accompanied by one or more moons; only Mercury and Venus move through space alone. There are more than 210 known moons orbiting planets and dwarf planets, and undoubtedly many other small ones remain undiscovered. The largest of the moons are as big as small planets and just as interesting. In addition to our Moon, the Solar System also contains the four largest moons of Jupiter (called the Galilean moons, after their discoverer) and the largest moons of Saturn and Neptune (confusingly named Titan and Triton, respectively).
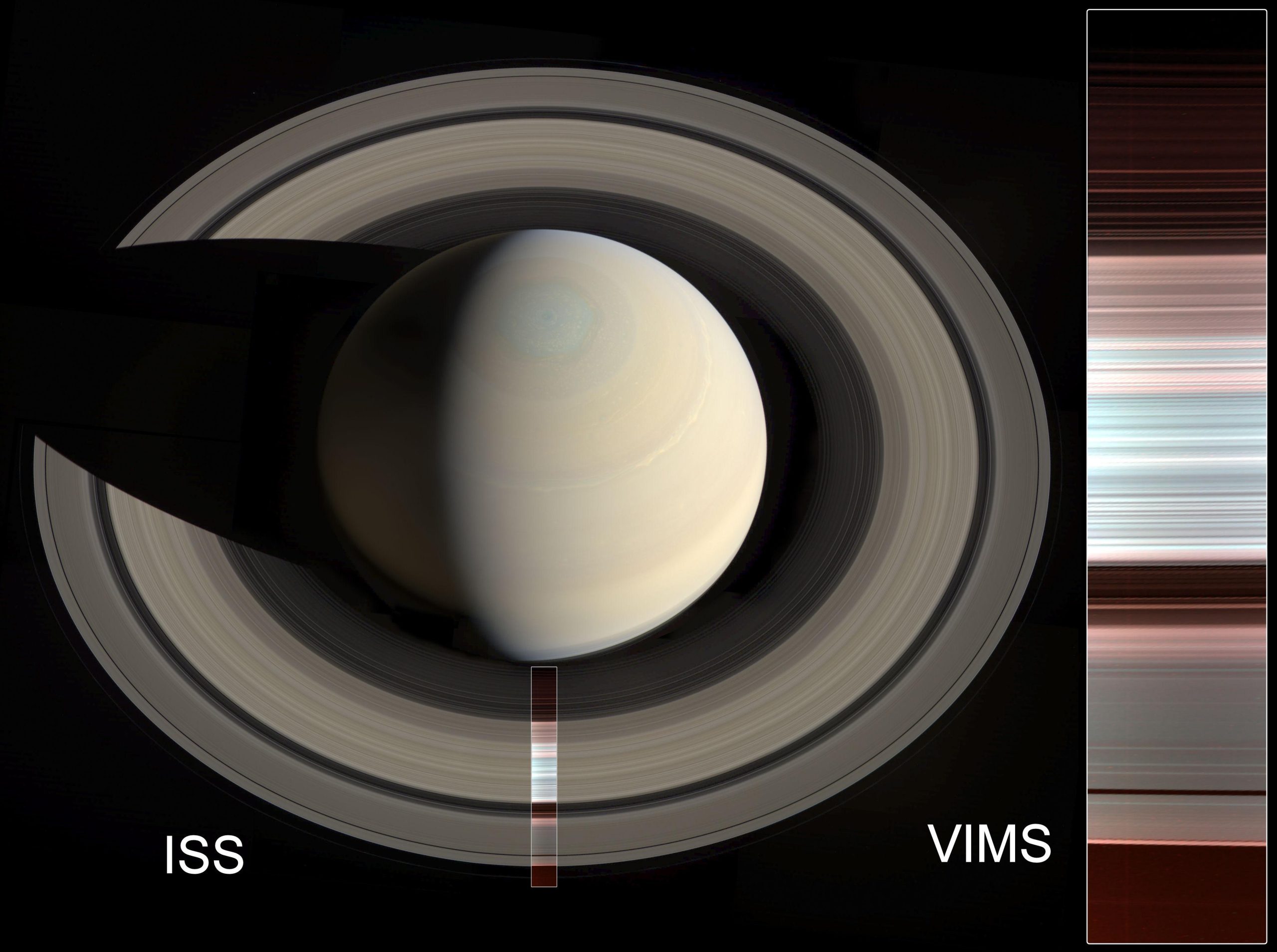
Each of the giant planets also has rings made up of countless small bodies ranging in size from mountains to mere grains of dust, all in orbit about the equator of the planet. The bright rings of Saturn are, by far, the easiest to see. They are among the most beautiful sights in the Solar System. However, all four ring systems are interesting to scientists because of their complicated forms, influenced by the pull of the moons that also orbit these giant planets.
The Solar System has many other less-conspicuous members. Another group is the asteroids, rocky bodies that orbit the Sun like miniature planets. Most asteroids are located in the Asteroid Belt, a region between the orbits of Mars and Jupiter (although some do cross the orbits of planets like Earth). A small percentage of asteroids are located outside the main Asteroid Belt. The Trojan asteroids travel along Jupiter’s orbit in two loose groups that orbit the Sun, with one group always ahead of Jupiter and the other always behind. Most asteroids are remnants of the initial population of the Solar System that existed before the planets themselves formed. Some of the smallest moons of the planets, such as the moons of Mars, are very likely captured asteroids.

Another class of small bodies is composed mostly of ice made of frozen gases such as water, carbon dioxide, and carbon monoxide; these objects are called comets. Comets are also remnants from the formation of the Solar System, but they were formed and continue (with rare exceptions) to orbit the Sun in distant, cooler regions—stored in a sort of cosmic deep freeze. This is also the realm of the larger icy worlds called dwarf planets.

Finally, there are countless grains of broken rock, which we call cosmic dust, scattered throughout the Solar System. When these particles enter Earth’s atmosphere (as millions do each day), they burn up, producing a brief flash of light in the night sky known as a meteor (meteors are often referred to as shooting stars). Occasionally, some larger chunk of rocky or metallic material survives its passage through the atmosphere and lands on Earth. Any piece that strikes the ground is known as a meteorite. You can see meteorites on display in many natural history museums and can sometimes even purchase pieces of them from gem and mineral dealers.
17.2.3 A Scale Model of the Solar System
Astronomy often deals with dimensions and distances that far exceed our ordinary experience. What does 1.4 billion kilometers—the distance from the Sun to Saturn—really mean to anyone? It can be helpful to visualize such large systems in terms of a scale model.
In our imaginations, let us build a scale model of the Solar System, adopting a scale factor of 1 billion (109)—that is, reducing the actual Solar System by dividing every dimension by a factor of 109. Earth, then, has a diameter of 1.3 centimeters, about the size of a grape. The Moon is a pea orbiting this at a distance of 40 centimeters, or a little more than a foot away. This Earth-Moon system fits into a standard backpack.
In this model, the Sun is nearly 1.5 meters in diameter, about the average height of an adult, and our Earth is at a distance of 150 meters—about one city block—from the Sun. Jupiter is five blocks away from the Sun, and its diameter is 15 centimeters, about the size of a very large grapefruit. Saturn is ten blocks from the Sun; Uranus, 20 blocks; and Neptune, 30 blocks. Pluto, with a distance that varies quite a bit during its 249-year orbit, is currently just beyond 30 blocks and getting farther with time. Most of the moons of the outer Solar System are the sizes of various kinds of seeds orbiting the grapefruit, oranges, and lemons that represent the outer planets.
In our scale model, a human is reduced to the dimensions of a single atom, and cars and spacecraft to the size of molecules. Sending the Voyager spacecraft to Neptune involves navigating a single molecule from the Earth-grape toward a lemon five kilometers away with an accuracy equivalent to the width of a thread in a spider’s web.
If that model represents the Solar System, where would the nearest stars be? If we keep the same scale, the closest stars would be tens of thousands of kilometers away. If you built this scale model in the city where you live, you would have to place the representations of these stars on the other side of Earth or beyond.
By the way, model solar systems like the one we just presented have been built in cities throughout the world. In Sweden, for example, Stockholm’s huge Globe Arena has become a model for the Sun, and Pluto is represented by a 12-centimeter sculpture in the small town of Delsbo, 300 kilometers away. Another model solar system is in Washington on the Mall between the White House and Congress (perhaps proving they are worlds apart?).
Take this quiz to check your comprehension of this section.
If you are using an offline version of this text, access the quiz for Section 17.2 via the QR code.
17.3 Composition and Structure of Planets
The fact that there are two distinct kinds of planets—the rocky terrestrial planets and the gas-rich Jovian planets—leads us to believe that they formed under different conditions. Certainly their compositions are dominated by different elements. Let us look at each type in more detail.
17.3.1 The Terrestrial Planets
The terrestrial planets are quite different from the giants. In addition to being much smaller, they are composed primarily of rocks and metals. These, in turn, are made of elements that are less common in the universe as a whole. On terrestrial planets, the most abundant minerals are silicates, which are composed of silicon and oxygen, and the most common metal is iron. We can tell from their densities that Mercury has the greatest proportion of metals (which are denser) and the Moon has the lowest. Earth, Venus, and Mars all have roughly similar bulk compositions; about one-third of their mass consists of iron-nickel or iron-sulfur combinations, and two-thirds of the mass is from silicates. Because these planets are largely composed of oxygen compounds (such as the silicate minerals of their crusts), their chemistry is said to be oxidized.
When we look at the internal structure of each of the terrestrial planets, we find that the densest metals are in a central core, with the lighter silicates near the surface. If these planets were liquid, like the giant planets, we could understand this effect as the result the sinking of heavier elements due to the pull of gravity. This leads us to conclude that, although the terrestrial planets are solid today, they must have been hot enough to melt at one time.
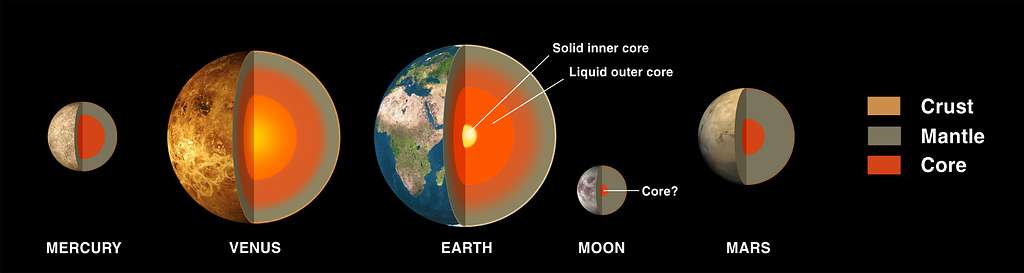
Differentiation is the process by which gravity helps separate a planet’s interior into layers of different compositions and densities. The heavier metals sink to form a core, while the lightest minerals float to the surface to form a crust. Later, when the planet cools, this layered structure is preserved. In order for a rocky planet to differentiate, it must be heated to the melting point of rocks, which is typically more than 1300 K.
17.3.2 The Giant Planets
The two largest planets, Jupiter and Saturn, have nearly the same chemical makeup as the Sun; they are composed primarily of the elements hydrogen and helium, with 75% of their mass being hydrogen and 25% helium. On Earth, both hydrogen and helium are gases, so Jupiter and Saturn are sometimes called gas planets. But this name is misleading. Jupiter and Saturn are so large that the gas is compressed in their interior until the hydrogen becomes a liquid. Because the bulk of both planets consists of compressed, liquefied hydrogen, we should really call them liquid planets.
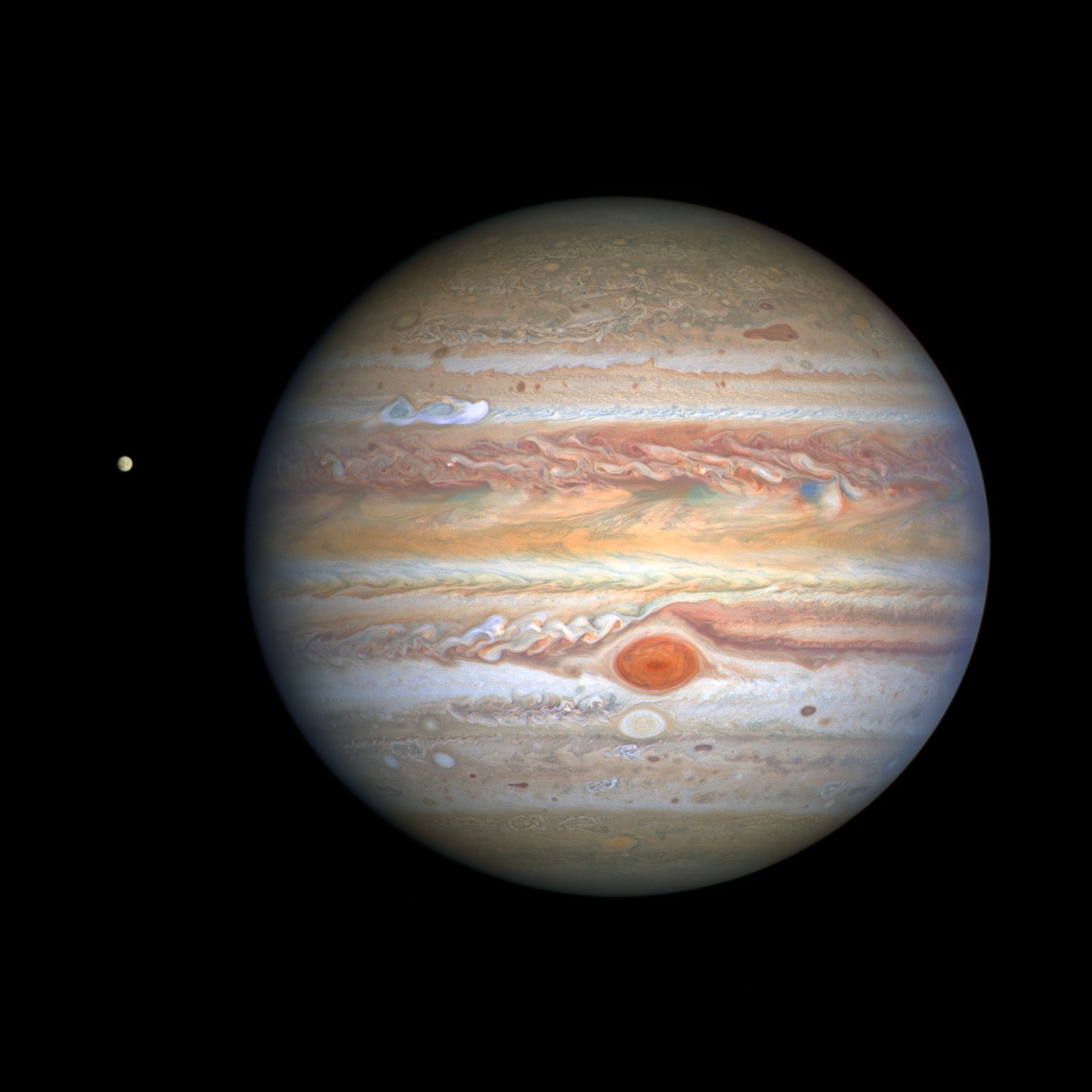
Under the force of gravity, the heavier elements sink toward the inner parts of a liquid or gaseous planet. Both Jupiter and Saturn, therefore, have cores composed of heavier rock, metal, and ice, but we cannot see these regions directly. We must infer the existence of the denser core inside these planets from studies of each planet’s gravity. When we look down at each giant planet from above, all we see is a thick atmosphere with swirling clouds. The rapid rotations of Jupiter and Saturn create extreme weather patterns in their atmospheres. Jupiter’s atmosphere, for example, features a counterclockwise rotating cyclonic storm in its southern hemisphere known as the Great Red Spot, which has been visible to humans for over 300 years.
Uranus and Neptune are much smaller than Jupiter and Saturn, but each also has a core of rock, metal, and ice. Uranus and Neptune were less efficient at attracting hydrogen and helium gas, so they have much smaller atmospheres in proportion to their cores.
Chemically, each giant planet is dominated by hydrogen and its many compounds. Nearly all the oxygen present is combined chemically with hydrogen to form water (H2O). Chemists call such a hydrogen-dominated composition reduced. Throughout the outer Solar System, we find abundant water (mostly in the form of ice) and reducing chemistry.
17.3.3 Moons, Asteroids, and Comets
Chemically and structurally, Earth’s Moon is like the terrestrial planets, but most moons are in the outer Solar System and have compositions similar to the cores of the giant planets around which they orbit. The four largest moons of Jupiter, known as the Galilean moons, are Ganymede, Callisto, Io, and Europa. They are named after the Italian astronomer Galileo Galilei, who first observed them through a telescope in 1610. The interiors of Ganymede, Io, and Europa have a layered structure. Io has a core, a mantle of partially molten rock, and a crust of solid rock coated with sulfur compounds. Both Europa and Ganymede have iron-rich cores, rocky mantles, and upper layers of water in both ice and liquid forms. Like Europa, Ganymede and Callisto have oceans, but these are deeper and less accessible than Europa’s and their seafloors are covered with thick layers of ice.
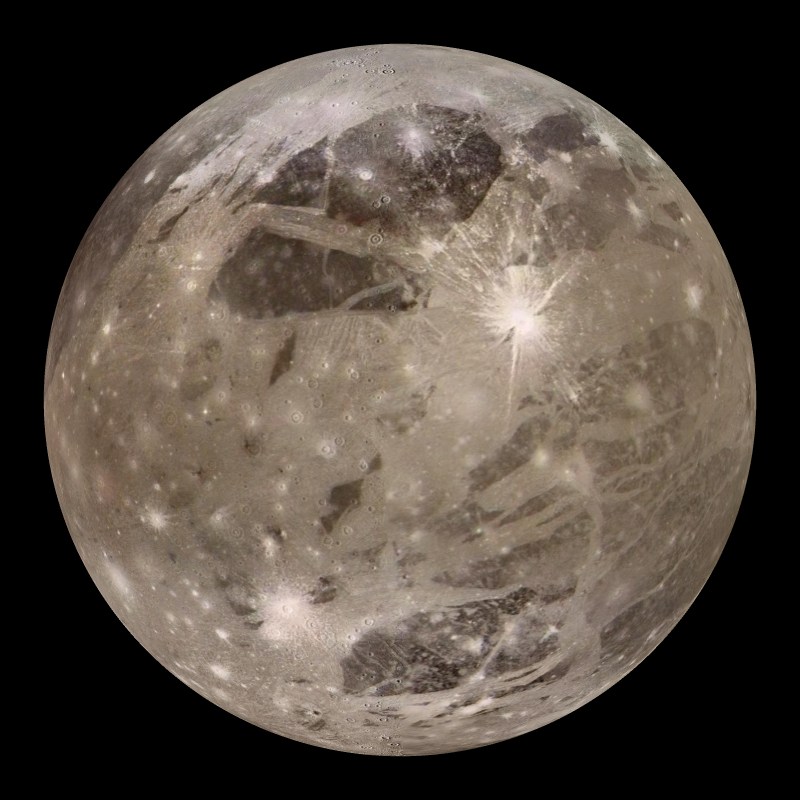
Most of the asteroids and comets, as well as the smallest moons, were probably never heated to the melting point. However, some of the largest asteroids, such as Vesta, appear to be differentiated; others are fragments from differentiated bodies. Many of the smaller objects seem to be fragments or rubble piles that are the result of collisions. Because most asteroids and comets retain their original compositions, they represent relatively unmodified material dating back to the time of the formation of the Solar System. In a sense, they act as chemical fossils, helping us to learn about a long-ago time whose traces have been erased.
17.3.4 Temperatures: Going to Extremes
Generally speaking, the farther a planet or moon is from the Sun, the cooler its surface. The planets are heated by the radiant energy of the Sun, which gets weaker with the square of the distance. You know how rapidly the heating effect of a fireplace or an outdoor radiant heater diminishes as you walk away from it; the same effect applies to the Sun. Mercury, the closest planet to the Sun, has a blistering surface temperature reaching 430°C on its sunlit side, whereas the surface temperature on Pluto is only about –220°C, colder than liquid air. Although it is the closest planet to the Sun, Mercury has no atmosphere to trap the heat, and thus the night side plunges to frigid lows of -180°C.
Mathematically, the temperatures decrease approximately in proportion to the square root of the distance from the Sun. Pluto is about 30 astronomical units (AU) at its closest to the Sun (or 100 times the distance of Mercury) and about 49 AU at its farthest from the Sun. Thus, Pluto’s temperature is less than that of Mercury by the square root of 100, or a factor of 10: from 500 K to 50 K.
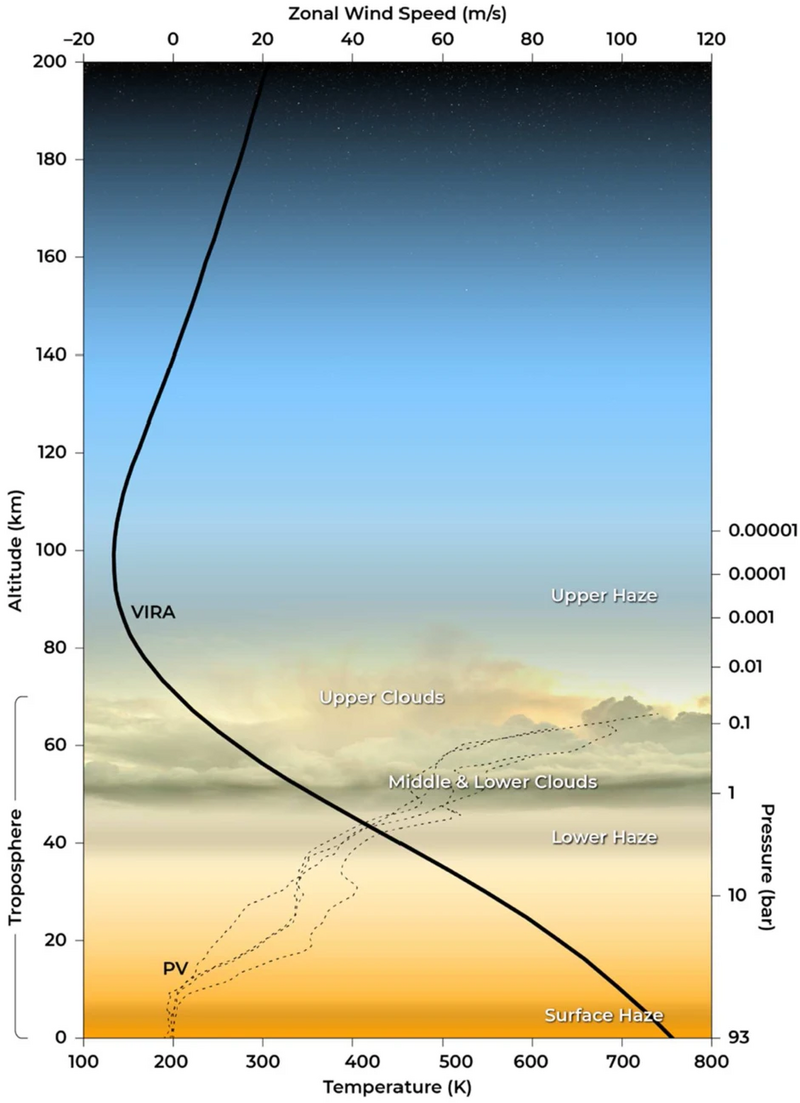
In addition to its distance from the Sun, the surface temperature of a planet can be influenced strongly by its atmosphere. The extreme temperature swings between day and night on Mercury are caused by its lack of atmosphere. Venus’s thick atmosphere of carbon dioxide acts as insulation, reducing the escape of heat built up at the surface, which results in an extreme greenhouse effect and temperatures greater than those on Mercury. Temperatures on Venus can reach 471°C, making it the hottest planet in our Solar System.
Today, Earth is the only planet where surface temperatures generally lie between the freezing and boiling points of water. Without our atmospheric insulation (the greenhouse effect, which keeps the heat in), the oceans of Earth would be permanently frozen. As far as we know, Earth is the only planet to support life.
Conversely, if Mars once had a larger atmosphere in the past, it could have supported a more temperate climate than it has today. Today, Mars has a very thin atmosphere, 1% as dense as Earth’s. Like Venus, it consists of mostly carbon dioxide along with small amounts of nitrogen, oxygen, and water vapor. However, due to the thin atmosphere, it cannot retain the heat trapped by atmospheric carbon dioxide. Consequently, Martian surface temperatures range from -153°C to 20°C.
17.3.5 Dating Planetary Surfaces
How do we know the age of the surfaces we see on planets and moons? If a world has a surface (as opposed to being mostly gas and liquid), astronomers have developed some techniques for estimating how long ago that surface solidified. Note that the age of these surfaces is not necessarily the age of the planet as a whole. On geologically active objects (including Earth), vast outpourings of molten rock or the erosive effects of water and ice, which we call planet weathering, have erased evidence of earlier epochs and present us with only a relatively young surface for investigation.
One way to estimate the age of a surface is by counting the number of impact craters. This technique works because the rate at which impacts have occurred in the Solar System has been roughly constant for several billion years. Thus, in the absence of forces to eliminate craters, the number of craters is simply proportional to the length of time the surface has been exposed. This technique has been applied successfully to many solid planets and moons.
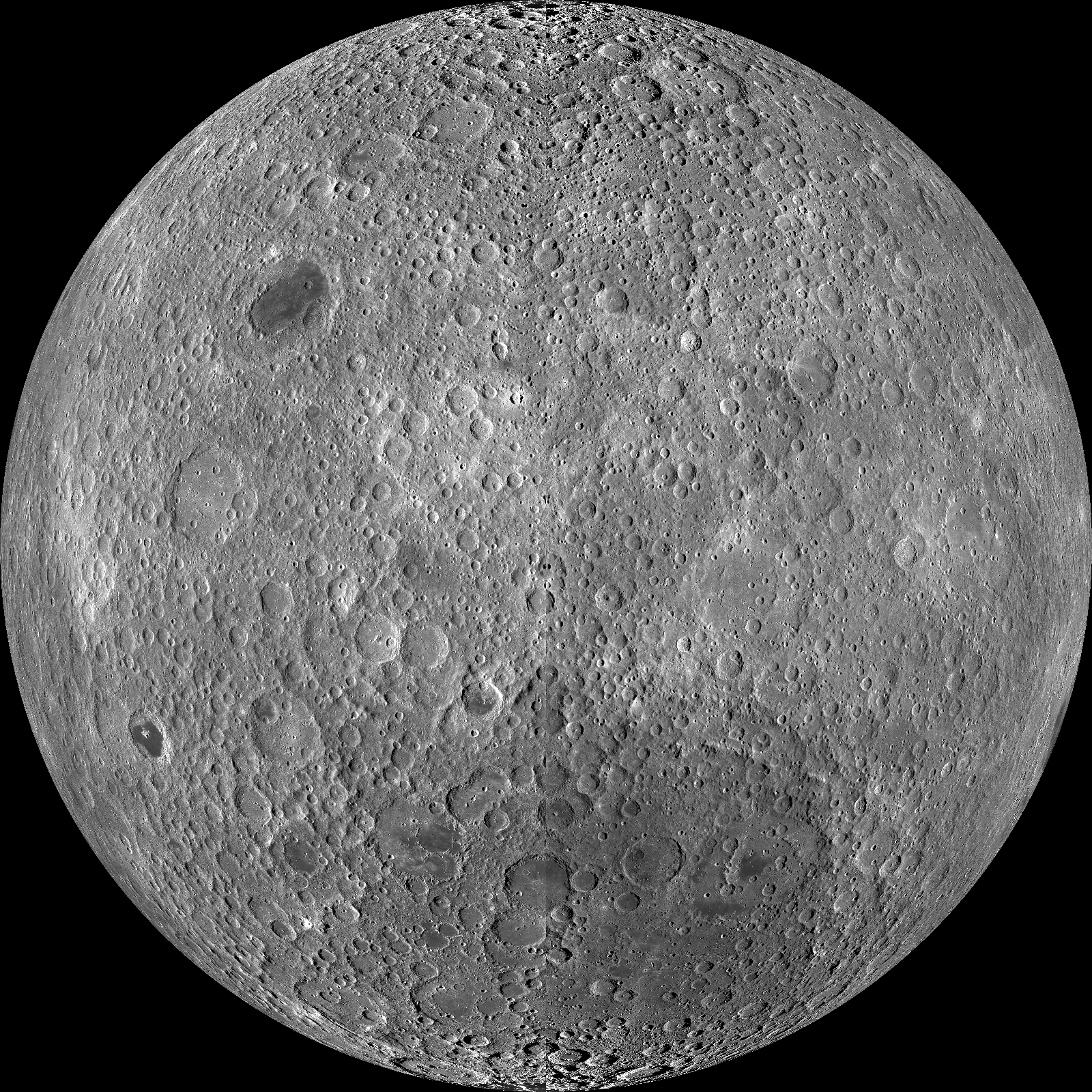
Bear in mind that crater counts can tell us only the time since the surface experienced a major change that could modify or erase preexisting craters. Estimating ages from crater counts is a little like walking along a sidewalk in a snowstorm after the snow has been falling steadily for a day or more. You may notice that the snow is deep in front of one house, while next door the sidewalk may be almost clear. Do you conclude that less snow has fallen in front of Ms. Jones’ house than Mr. Smith’s? More likely, you conclude that Jones has recently swept the walk clean and Smith has not. Similarly, the numbers of craters indicate how long it has been since a planetary surface was last “swept clean” by ongoing lava flows or by molten materials ejected when a large impact happened nearby.
Still, astronomers can use the numbers of craters on different parts of the same world to provide important clues about how regions on that world evolved. On a given planet or moon, the more heavily cratered terrain will generally be older (that is, more time will have elapsed there since something swept the region clean).
17.3.6 Radioactive Rocks
Another way to trace the history of a solid world is to measure the age of individual rocks. After samples were brought back from the Moon by Apollo astronauts, the techniques that had been developed to date rocks on Earth were applied to rock samples from the Moon to establish a geological chronology for the Moon. Furthermore, a few samples of material from the Moon, Mars, and the large asteroid Vesta have fallen to Earth as meteorites and can be examined directly.
Scientists measure the age of rocks using the properties of natural radioactivity. Around the beginning of the twentieth century, physicists began to understand that some atomic nuclei are not stable but can split apart (decay) spontaneously into smaller nuclei. The process of radioactive decay involves the emission of particles such as electrons or of radiation in the form of gamma rays.
For any one radioactive nucleus, it is not possible to predict when the decay process will happen. Such decay is random in nature, like the throw of dice: as gamblers have found all too often, it is impossible to say when the dice will come up 7 or 11. But, for a very large number of dice tosses, we can calculate the odds that 7 or 11 will come up. Similarly, if we have a very large number of radioactive atoms of one type, there is a specific time period, called its half-life, during which the chances are fifty-fifty that decay will occur for any of the nuclei.
A particular nucleus may last a shorter or longer time than its half-life, but in a large sample, almost exactly half of the nuclei will have decayed after a time equal to one half-life. Half of the remaining nuclei will have decayed after two half-lives pass, leaving only one half of a half—or one quarter—of the original sample.
If you had one gram of pure radioactive nuclei with a half-life of 100 years, then after 100 years you would have 1/2 gram; after 200 years, 1/4 gram; after 300 years, only 1/8 gram; and so forth. However, the material does not disappear. Instead, the radioactive atoms are replaced with their decay products. Sometimes the radioactive atoms are called parents and the decay products are called daughter elements.
In this way, radioactive elements with half-lives we have determined can provide accurate nuclear clocks. By comparing how much of a radioactive parent element is left in a rock to how much of its daughter products have accumulated, we can learn how long the decay process has been going on and hence how long ago the rock formed. The following table summarizes the decay reactions used most often to date lunar and terrestrial rocks.
Parent | Daughter | Half-life (billions of years) |
---|---|---|
Samarium-147 | Neodymium-143 | 106 |
Rubidium-87 | Strontium-87 | 48.8 |
Thorium-232 | Lead-208 | 14 |
Uranium-238 | Lead-206 | 4.47 |
Potassium-40 | Argon-40 | 1.31 |
Table 17.3: Radioactive decay reaction used to date rocks. The number after each element is its atomic weight equal to the number of protons plus neutrons in its nucleus. This specifies the isotope of the element, as different isotopes of the same element differ in the number of neutrons.
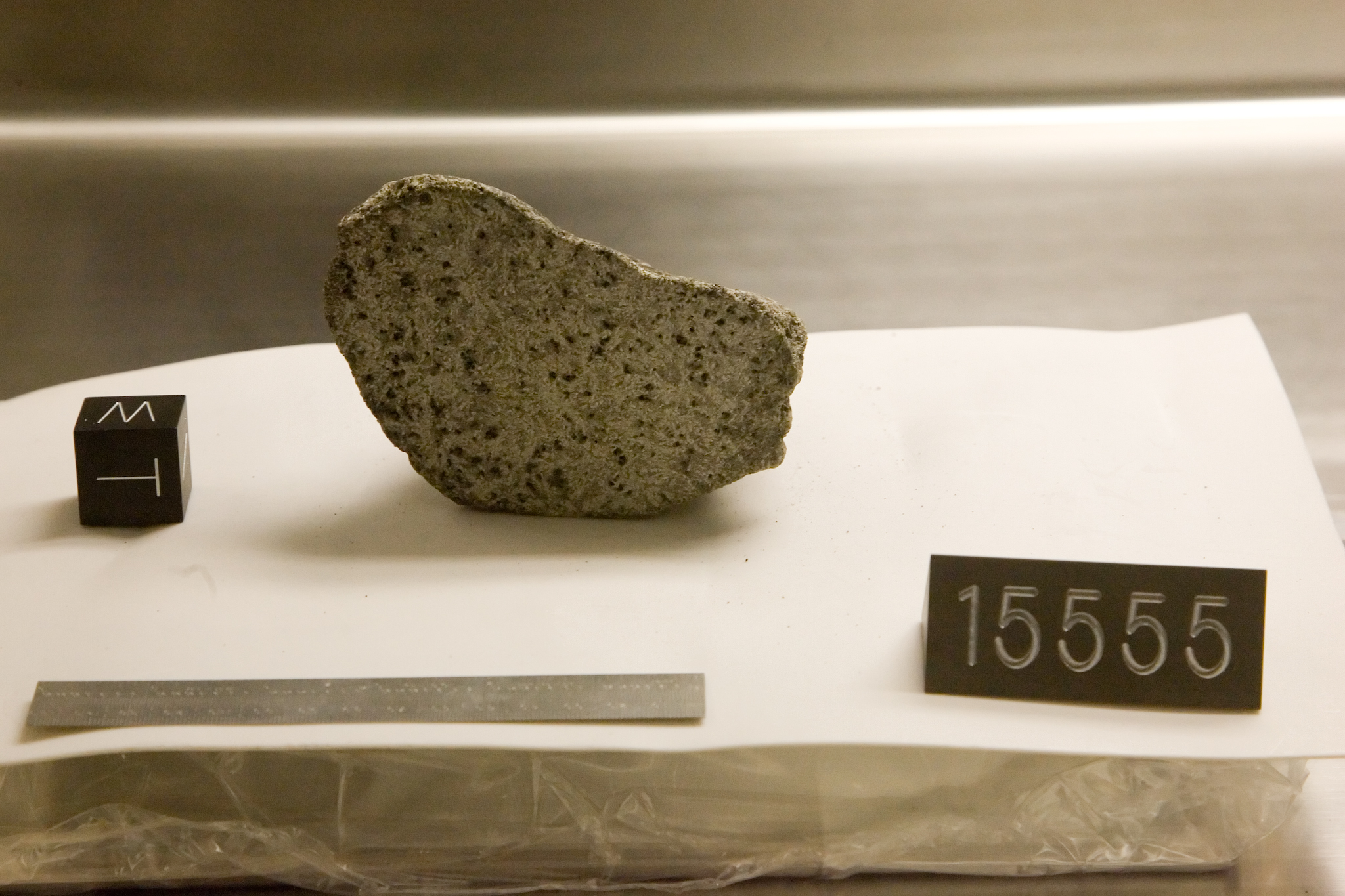
When astronauts first flew to the Moon, one of their most important tasks was to bring back lunar rocks for radioactive age-dating. Until then, astronomers and geologists had no reliable way to measure the age of the lunar surface. Counting craters had let us calculate relative ages (for example, the heavily cratered lunar highlands were older than the dark lava plains), but scientists could not measure the actual age in years. Some thought that the ages were as young as those of Earth’s surface, which has been resurfaced by many geological events. The Moon’s surface being so young would imply active geology on our satellite. Only in 1969, when the first Apollo samples were dated, did we learn that the Moon is an ancient, geologically dead world. Using such dating techniques, we have been able to determine the ages of both Earth and the Moon; each was formed about 4.5 billion years ago (although, as we shall see, Earth probably formed earlier than the Moon). Similarly, radioactive dating of meteorites allowed scientists to determine that the Solar System itself is nearly 4.6 billion years old.
We should also note that the decay of radioactive nuclei generally releases energy in the form of heat. Although the energy from a single nucleus is not very large (in human terms), the enormous numbers of radioactive nuclei in a planet or moon (especially early in its existence) can be a significant source of internal energy for that world. Geologists estimate that about half of Earth’s current internal heat budget comes from the decay of radioactive isotopes in its interior.
Take this quiz to check your comprehension of this section.
If you are using an offline version of this text, access the quiz for Section 17.3 via the QR code.
17.4 Origin of the Solar System
Much of astronomy is motivated by a desire to understand the origin of things—to find at least partial answers to age-old questions of where the universe, the Sun, Earth, and we ourselves came from. Each planet and moon is a fascinating place that may stimulate our imagination as we try to picture what it would be like to visit. Taken together, the members of the Solar System preserve patterns that can tell us about the formation of the entire system. As we begin our exploration of the planets, we want to introduce our modern picture of how the Solar System formed.
The recent discovery of thousands of planets in orbit around other stars has shown astronomers that many exoplanetary systems can be quite different from our own Solar System. For example, it is common for these systems to include planets intermediate in size between our terrestrial and giant planets. These are often called superearths. Some exoplanet systems even have giant planets close to the star, reversing the order we see in our system.
17.4.1 Looking for Patterns
One way to approach our question of origin is to look for regularities among the planets. We found, for example, that all the planets lie in nearly the same plane and revolve in the same direction around the Sun. The Sun also spins in the same direction about its own axis. Astronomers interpret this pattern as evidence that the Sun and planets formed together from a spinning cloud of gas and dust that we call the solar nebula.
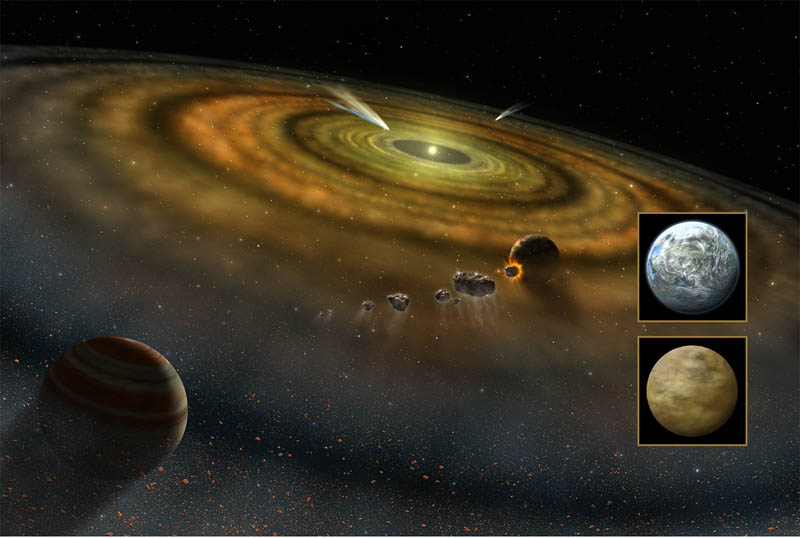
The composition of the planets gives another clue about origins. Spectroscopic analysis allows us to determine which elements are present in the Sun and the planets. The Sun has the same hydrogen-dominated composition as Jupiter and Saturn, and therefore it appears to have been formed from the same reservoir of material. In comparison, the terrestrial planets and our Moon are relatively deficient in the light gases and the various ices that form from the common elements oxygen, carbon, and nitrogen. Instead, on Earth and its neighbors, we see mostly the rarer heavy elements such as iron and silicon. This pattern suggests that the processes that led to planet formation in the inner Solar System must somehow have excluded much of the lighter materials that are common elsewhere. These lighter materials must have escaped, leaving a residue of heavy stuff.
The reason for this is not hard to guess, bearing in mind the heat of the Sun. The inner planets and most of the asteroids are made of rock and metal, which can survive heat, but they contain very little ice or gas, which evaporate when temperatures are high (to see what we mean, just compare how long a rock and an ice cube survive when they are placed in the sunlight). In the outer Solar System, where it has always been cooler, the planets and their moons are mostly composed of ice and gas, as are dwarf planets and comets.
17.4.2 The Evidence from Far Away
A second approach to understanding the origins of the Solar System is to look outward for evidence that other systems of planets are forming elsewhere. We cannot look back in time to the formation of our own system, but many stars in space are much younger than the Sun. In these systems, the processes of planet formation might still be accessible to direct observation. We observe that there are many other solar nebulas or circumstellar disks—flattened, spinning clouds of gas and dust surrounding young stars. These disks resemble our own Solar System’s initial stages of formation billions of years ago.
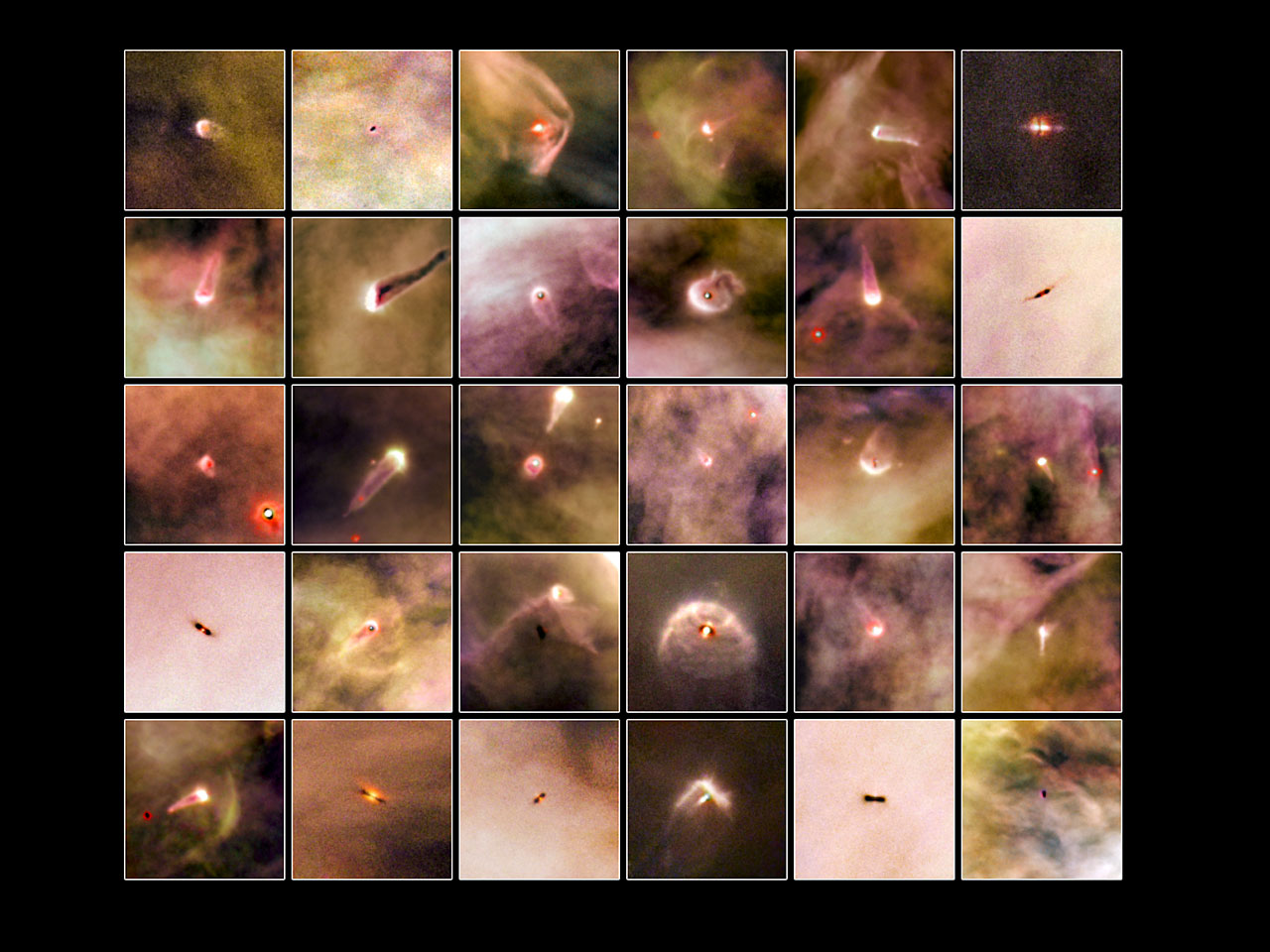
17.4.3 Building Planets
Circumstellar disks are a common occurrence around very young stars, suggesting that disks and stars form together. Astronomers can use theoretical calculations to see how solid bodies might form from the gas and dust in these disks as they cool. These models show that material begins to coalesce first by forming smaller objects, precursors of planets, which we call planetesimals.
Today’s fast computers can simulate the way millions of planetesimals, probably no larger than 100 kilometers in diameter, might gather together under their mutual gravity to form the planets we see today. We are beginning to understand that this process was a violent one, with planetesimals crashing into each other and sometimes even disrupting the growing planets themselves. As a consequence of those violent impacts (and the heat from radioactive elements in them), all the planets were heated until they were liquid and gas and were therefore differentiated, which helps explain their present internal structures.
The process of impacts and collisions in the early Solar System was complex and, apparently, often random. The solar nebula model can explain many of the regularities we find in the Solar System, but the random collisions of massive collections of planetesimals could be the reason for some exceptions to the “rules” of solar system behavior. For example, why do the planets Uranus and Pluto spin on their sides? Why does Venus spin slowly and in the opposite direction from the other planets? Why does the composition of the Moon resemble Earth in many ways and yet exhibit substantial differences? The answers to such questions probably lie in enormous collisions that took place in the solar system long before life on Earth began.
Today, some 4.5 billion years after its origin, the Solar System is—thank goodness—a much less violent place. However, some planetesimals have continued to interact and collide, and their fragments move about the Solar System as roving “transients” that can make trouble for the established members of the Sun’s family, such as our own Earth.
Take this quiz to check your comprehension of this section.
If you are using an offline version of this text, access the quiz for Section 17.4 via the QR code.
17.5 The Search for Life in the Universe
As we have learned more about the universe, we have naturally wondered whether there might be other forms of life out there. The ancient question “Are we alone in the universe?” connects us to generations of humans before us. While in the past, this question was in the realm of philosophy or science fiction, today we have the means to seek an answer through scientific inquiry. In this chapter, we will consider how life began on Earth, whether the same processes could have led to life on other worlds, and how we might seek evidence of life elsewhere. This is the science of astrobiology.
The search for life on other planets is not the same as the search for intelligent life, which (if it exists) is surely much rarer. Learning more about the origin, evolution, and properties of life on Earth aids us in searching for evidence of all kinds of life beyond that on our planet.
Astronomers and planetary scientists continue to search for life in the Solar System and the universe at large, with their searches falling into two categories. First is the direct exploration of planets within our own solar system, especially Mars and some of the icy moons of the outer Solar System. Second is the even more difficult task of searching for evidence of life—a biomarker—on planets circling other stars. As you will see, the approaches taken are very different, even though the goal of each is the same: to determine if life on Earth is unique in the universe.
17.5.1 The Cosmic Context for Life
As the universe aged following the big bang, processes within stars created the other elements, including those that make up Earth (such as iron, silicon, magnesium, and oxygen) and those required for life as we know it (such as carbon, oxygen, and nitrogen). These and other elements combined in space to produce a wide variety of compounds that form the basis of life on Earth.
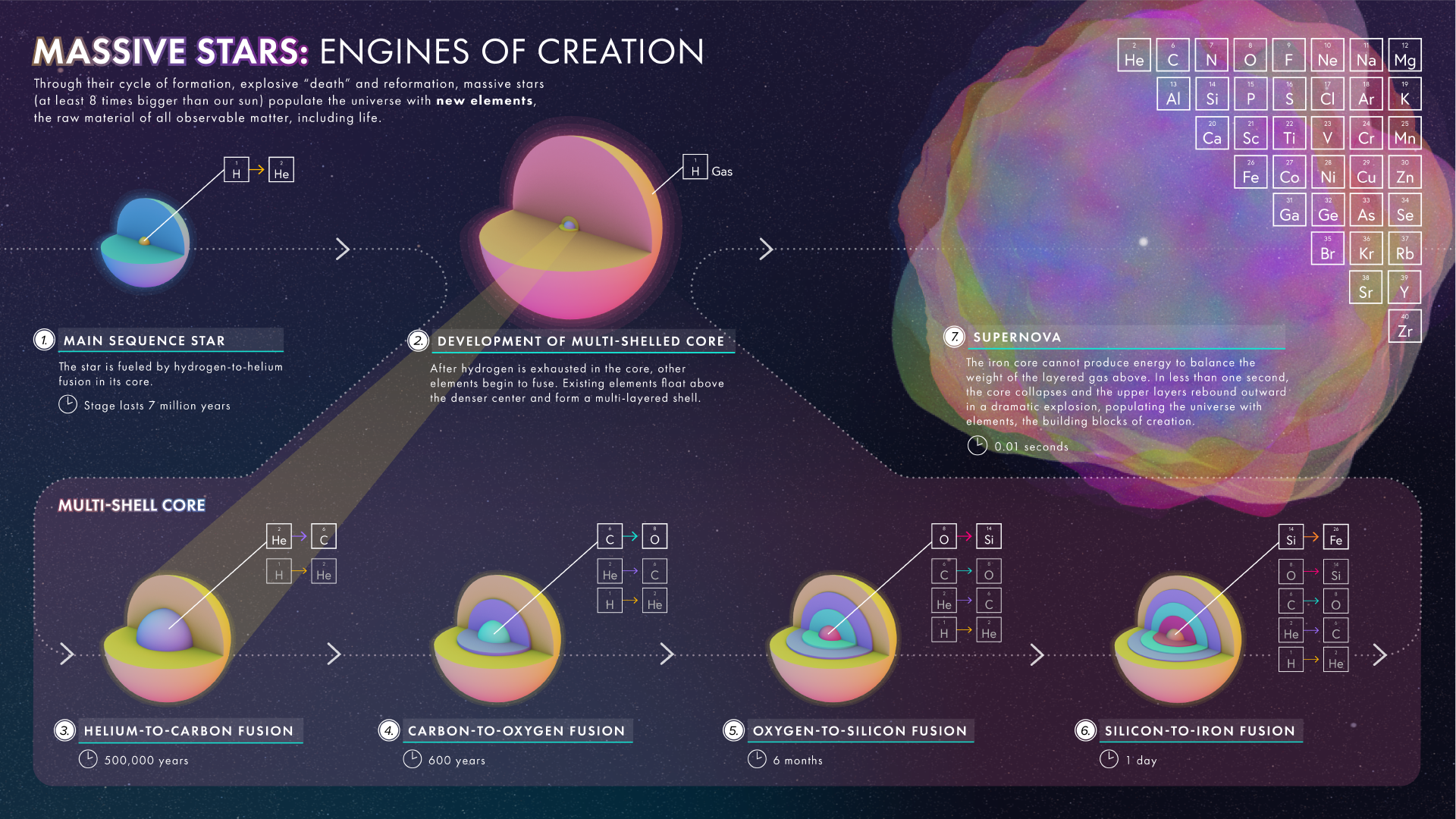
In particular, life on Earth is based on the presence of a key unit known as an organic molecule, a molecule that contains carbon. Especially important are the hydrocarbons, chemical compounds made up entirely of hydrogen and carbon, which serve as the basis for our biological chemistry, or biochemistry. While we do not understand the details of how life on Earth began, it is clear that, to make creatures like us possible, events like those described above must have occurred, resulting in what is called the chemical evolution of the universe.
The chemical variety and moderate conditions on Earth eventually led to the formation of molecules that could make copies of themselves (reproduce), which is essential for beginning life. Over the billions of years of Earth history, life evolved and became more complex. The course of evolution was punctuated by occasional planet-wide changes caused by collisions with some of the smaller bodies that did not make it into the Sun or one of its accompanying worlds. In fact, mammals may owe their domination of Earth’s surface to just such a collision 65 million years ago, which led to the extinction of the dinosaurs (along with the majority of other living things). The details of such mass extinctions are currently the focus of a great deal of scientific interest.
Philosophers of science sometimes call the idea that there is nothing special about our place in the universe the Copernican principle. Following this principle, most scientists would be surprised if life were limited to our planet and had started nowhere else. There are billions of stars in our galaxy old enough for life to have developed on a planet around them, and there are billions of other galaxies as well. Astronomers and biologists have long conjectured that a series of events similar to those on the early Earth probably led to living organisms on many planets around other stars and possibly even on other bodies within our Solar System, such as Mars or the moon Europa.
The real scientific issue is whether organic biochemistry is likely or unlikely in the universe at large. Are we a fortunate and exceedingly rare outcome of chemical evolution, or is organic biochemistry a regular part of the chemical evolution of the cosmos? We do not yet know the answer to this question, but data, even an exceedingly small amount (like finding “unrelated to us” living systems on a world like Europa), will help us arrive at it.
17.5.2 Life on Mars
The possibility that Mars hosts (or has hosted) life has a rich history dating back to the “canals” that some people claimed to see on the Martian surface toward the end of the nineteenth century and the beginning of the twentieth. With the dawn of the Space Age came the possibility to address this question up close through a progression of missions to Mars that began with the first successful flyby of a robotic spacecraft in 1964 and have led to the deployment of capable rovers, like Curiosity and Perseverance, with instruments to look for organic chemistry.
The earliest missions to Mars provided some hints that liquid water—one of life’s primary requirements—may once have flowed on the surface, and later missions have strengthened this conclusion.
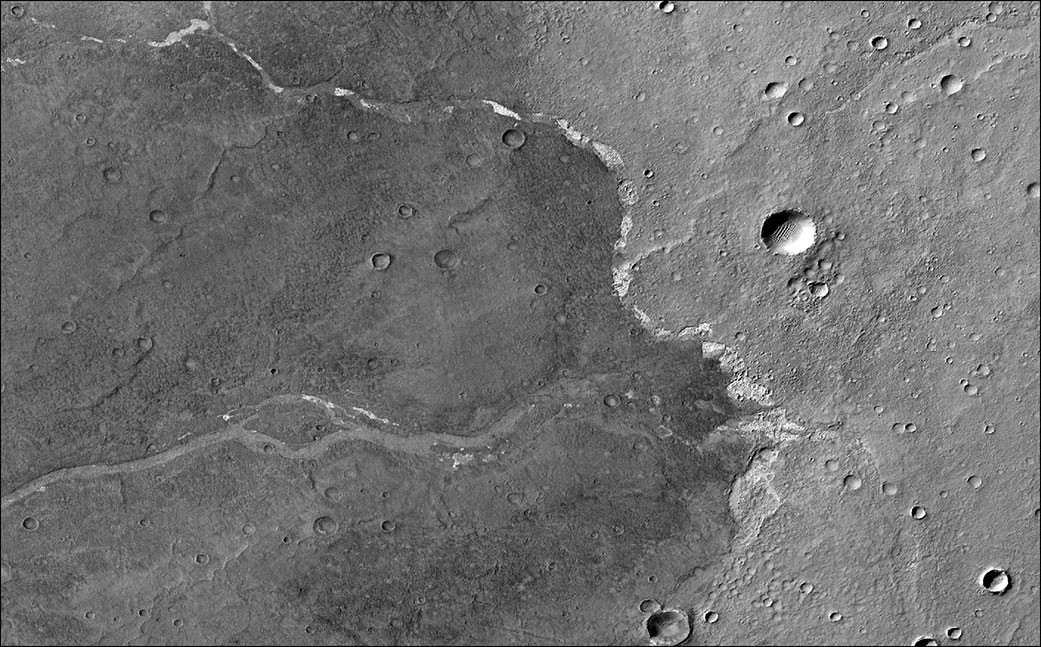
The NASA Viking landers, whose purpose was to search directly for evidence of life on Mars, arrived on Mars in 1976. Viking’s onboard instruments found no organic molecules (the stuff of which life is made), and no evidence of biological activity in the Martian soils it analyzed.
This result is not particularly surprising because, despite the evidence of flowing liquid water in the past, liquid water on the surface of Mars is generally not stable today. Over much of Mars, temperatures and pressures at the surface are so low that pure water would either freeze or boil away (under very low pressures, water will boil at a much lower temperature than usual). To make matters worse, unlike Earth, Mars has neither a magnetic field nor ozone layer to protect the surface from harmful solar ultraviolet radiation and energetic particles. However, Viking’s analyses of the soil said nothing about whether life may have existed in Mars’ distant past, when liquid water was more abundant. We do know that water in the form of ice exists in abundance on Mars, not so deep beneath its surface. Water vapor is also a constituent of the atmosphere of Mars.
Since the visit of Viking, our understanding of Mars has deepened spectacularly. Orbiting spacecraft have provided increasingly detailed images of the surface and detected the presence of minerals that could have formed only in the presence of liquid water. Multiple surface missions, including the Mars Exploration Rovers Spirit and Opportunity (2004), followed by the much larger Curiosity rover (2012) and Perseverance rover (2021), confirmed these remote-sensing data.
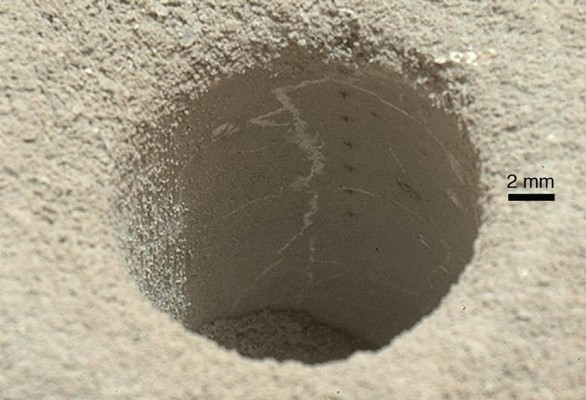
All of the rovers found abundant evidence for a past history of liquid water, revealed not only from the mineralogy of rocks they analyzed but also from the unique layering of rock formations. Curiosity has gone a step beyond evidence for water and confirmed the existence of habitable environments on ancient Mars. “Habitable” means not only that liquid water was present, but that life’s requirements for energy and elemental raw materials could also have been met.
17.5.3 Life in the Outer Solar System
The massive gas and ice giant planets of the outer Solar System—Jupiter, Saturn, Uranus, and Neptune—are almost certainly not habitable for life as we know it, but some of their moons might be. Although these worlds in the outer Solar System contain abundant water, they receive so little warming sunlight in their distant orbits that it was long believed they would be “geologically dead” balls of hard, frozen ice and rock. However, missions to the outer Solar System have found something much more interesting.
Jupiter’s moon Europa revealed itself to the Voyager and Galileo missions as an active world whose icy surface apparently conceals an ocean with a depth of tens to perhaps a hundred kilometers. As the moon orbits Jupiter, the planet’s massive gravity creates tides on Europa—just as our own Moon’s gravity creates our ocean tides—and the friction of all that pushing and pulling generates enough heat to keep the water in liquid form. Similar tides act upon other moons if they orbit close to the planet.
Europa has probably had an ocean for most or all of its history, but habitability requires more than just liquid water. Life also requires energy, and because sunlight does not penetrate below the kilometers-thick ice crust of Europa, this would have to be chemical energy.
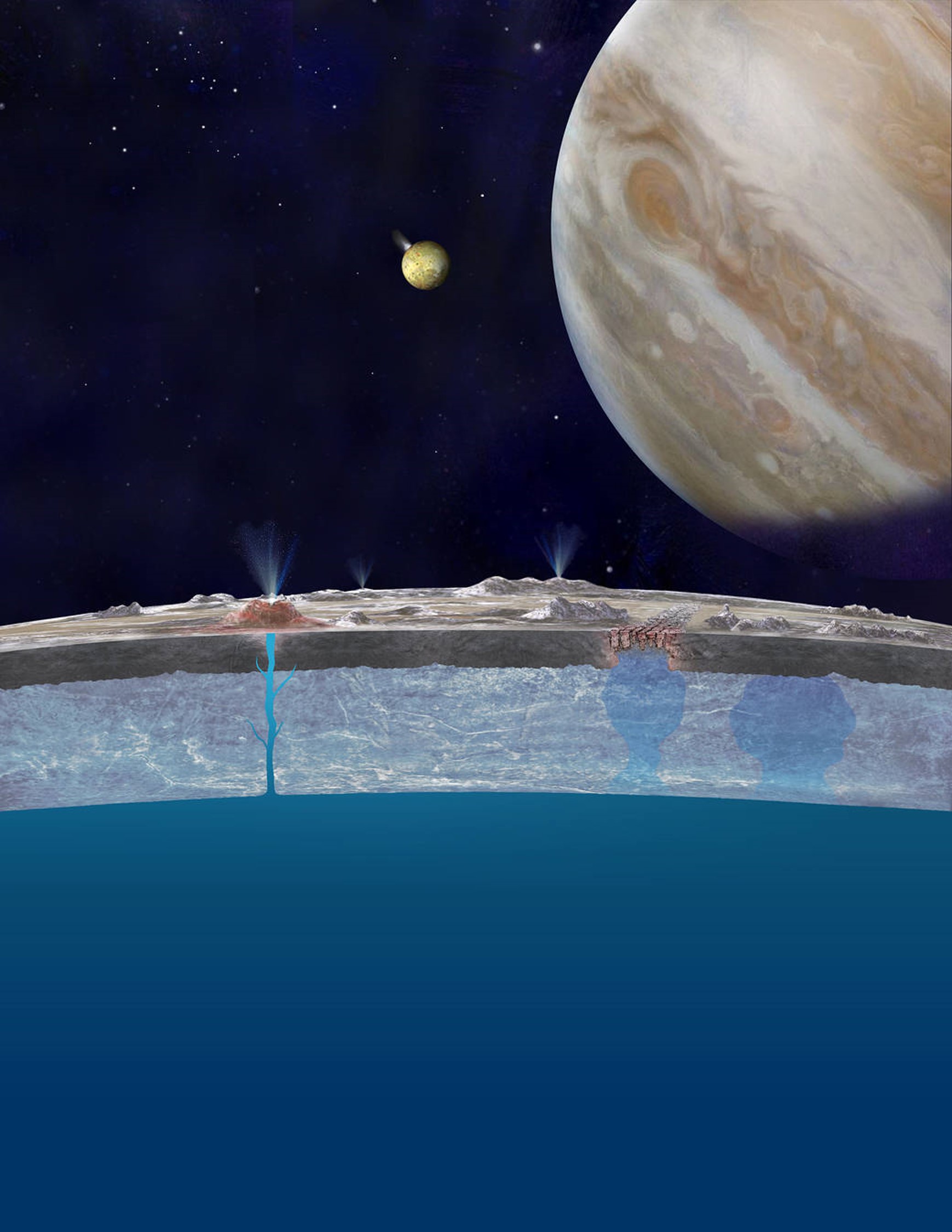
One of Europa’s key attributes from an astrobiology perspective is that its ocean is most likely in direct contact with an underlying rocky mantle, and the interaction of water and rocks—especially at high temperatures, as within Earth’s hydrothermal vent systems—yields a reducing chemistry (where molecules tend to give up electrons readily) that is like half of a chemical battery. To complete the battery and provide energy that could be used by life requires the availability of an oxidizing chemistry (where molecules tend to accept electrons readily). On Earth, when chemically reducing vent fluids meet oxygen-containing seawater, the energy that becomes available often supports thriving communities of microorganisms and animals on the seafloor, far from the light of the Sun.
In 2005, the Cassini mission performed a close flyby of a small (500-kilometer diameter) moon of Saturn, Enceladus, and made a remarkable discovery. Plumes of gas and icy material were venting from the moon’s south polar region at a collective rate of about 250 kilograms of material per second. Several observations, including the discovery of salts associated with the icy material, suggest that their source is a liquid water ocean beneath tens of kilometers of ice. Although it remains to be shown definitively whether the ocean is local or global, transient or long-lived, it does appear to be in contact with and have reacted to a rocky interior. As on Europa, this is probably a necessary—though not sufficient—condition for habitability. What makes Enceladus so enticing to planetary scientists, though, are those plumes of material that seem to come directly from its ocean: samples of the interior are there for the taking by any spacecraft sent flying through. For a future mission, such samples could yield evidence not only of whether Enceladus is habitable but, indeed, of whether it is home to life.
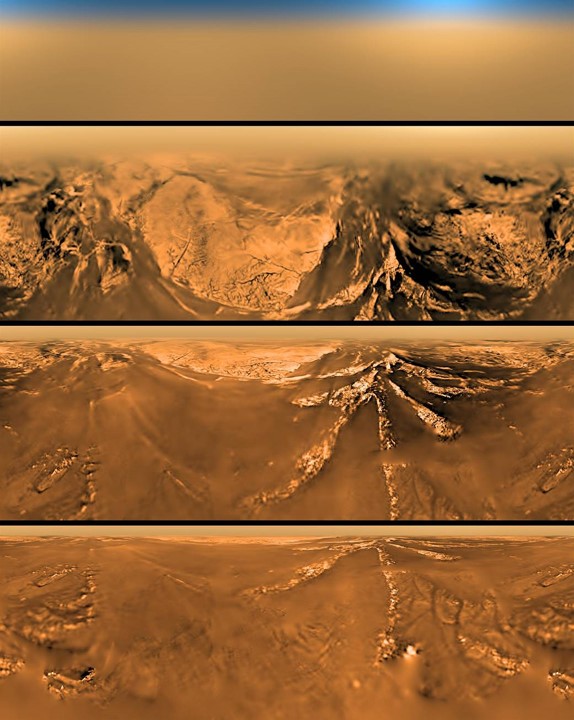
Saturn’s big moon Titan is very different from both Enceladus and Europa. Although it may host a liquid water layer deep within its interior, it is the surface of Titan and its unusual chemistry that makes this moon such an interesting place.
Titan’s thick atmosphere is composed mostly of nitrogen but also of about 5% methane. In the upper atmosphere, the Sun’s ultraviolet light breaks apart and recombines these molecules into more complex organic compounds that are collectively known as tholins. The tholins shroud Titan in an orange haze, and imagery from Cassini and from the Huygens probe that descended to Titan’s surface show that heavier particles appear to accumulate on the surface, even forming “dunes” that are cut and sculpted by flows of liquid hydrocarbons (such as liquid methane). Some scientists see this organic chemical factory as a natural laboratory that may yield some clues about the Solar System’s early chemistry—perhaps even chemistry that could support the origin of life.
17.5.4 Habitable Planets Orbiting Other Stars
One of the most exciting developments in astronomy during the last two decades is the ability to detect exoplanets—planets orbiting other stars. Since the discovery of the first exoplanet in 1995, there have been thousands of confirmed detections and many more candidates that are not yet confirmed. These include several dozen possibly habitable exoplanets. Such numbers finally allow us to make some predictions about exoplanets and their life-hosting potential. The majority of stars with mass similar to the Sun appear to host at least one planet, with multi-planet systems like our own not unusual. How many of these planets might be habitable, and how could we search for life there?
In evaluating the prospect for life in distant planetary systems, astrobiologists have developed the idea of a habitable zone—a region around a star where suitable conditions might exist for life. This concept focuses on life’s requirement for liquid water, and the habitable zone is generally thought of as the range of distances from the central star in which water could be present in liquid form at a planet’s surface. In our own Solar System, for example, Venus has surface temperatures far above the boiling point of water and Mars has surface temperatures that are almost always below the freezing point of water. Earth, which orbits between the two, has a surface temperature that is “just right” to keep much of our surface water in liquid form.

Whether surface temperatures are suitable for maintaining liquid water depends on a planet’s “radiation budget”—how much starlight energy it absorbs and retains—and whether or how processes like winds and ocean circulation distribute that energy around the planet. How much stellar energy a planet receives, in turn, depends on how much and what sort of light the star emits, how far the planet is from that star, how much it reflects back to space, and how effectively the planet’s atmosphere can retain heat through the greenhouse effect. All of these can vary substantially, and all matter a lot. We must consider the nature of any atmosphere as well as the distance from the star in evaluating the range of habitability.
Even when planets orbit within the habitable zone of their star, it is no guarantee that they are habitable. For example, Venus today has virtually no water, so even if it were suddenly moved to a “just right” orbit within the habitable zone, a critical requirement for life would still be lacking.
Scientists are working to understand all the factors that define the habitable zone and the habitability of planets orbiting within that zone; such an understanding will be our primary guide in targeting exoplanets on which to seek evidence of life. As technology for detecting exoplanets has advanced, so too has our potential to find Earth-size worlds within the habitable zones of their parent stars. Of the confirmed or candidate exoplanets known at the time of writing, nearly 300 are considered to be orbiting within the habitable zone and more than 10% of those are roughly Earth-size.
17.5.5 Communicating with the Stars
It seems likely that life could have developed on many planets around other stars. Even if that life is microbial, we may soon have ways to search for chemical biosignatures. This search is of fundamental importance for understanding biology, but it does not answer the question “Are we alone?” When we ask this question, many people think of other intelligent creatures, perhaps beings that have developed technology similar to our own. If any intelligent, technical civilizations have arisen, as has happened on Earth in the most recent blink of cosmic time, how could we make contact with them?
If we want to get in touch with intelligent life around other stars, we could travel or we could try to exchange messages. Because of the great distances involved, interstellar space travel would be very slow and prohibitively expensive. The fastest spacecraft the human species has built so far would take almost 80,000 years to get to the nearest star. While we could certainly design a faster craft, the more quickly it must travel, the greater the energy cost involved. To reach neighboring stars in less than a human life span, we would have to travel close to the speed of light.
While space travel by living creatures seems very difficult, robot probes can travel over long distances and over long periods of time. Five spacecraft—two Pioneers, two Voyagers, and New Horizons—are leaving the Solar System. At their coasting speeds, they will take hundreds of thousands or millions of years to get anywhere close to another star. On the other hand, they were the first products of human technology to go beyond our home system, so we wanted to put messages on board to show where they came from. Each Pioneer carries a plaque with a pictorial message engraved on a gold-anodized aluminum plate. The Voyagers, launched in 1977, have audio and video records attached, which allowed the inclusion of over 100 photographs and a selection of music from around the world.
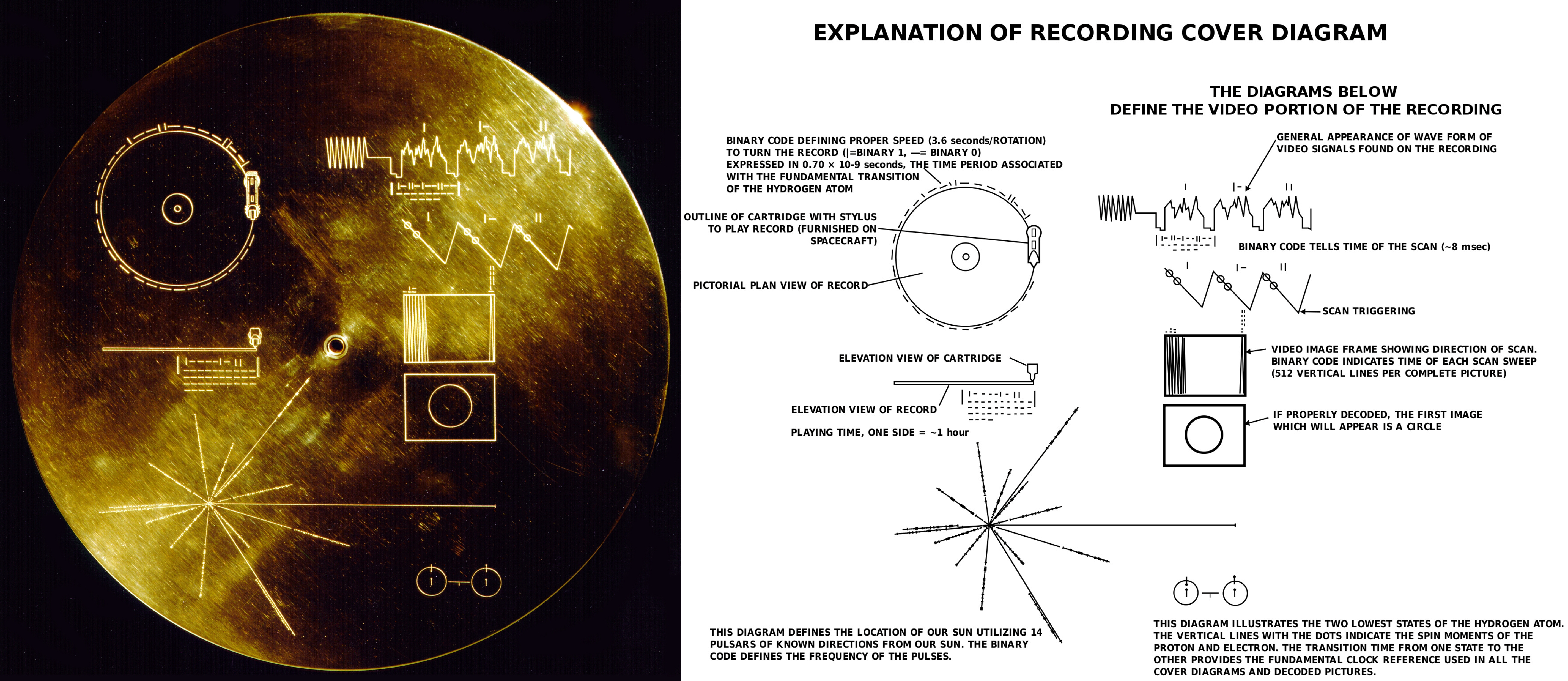
Given the enormous space between stars in our section of the galaxy, it is very unlikely that these messages will ever be received by anyone. They are more like a note in a bottle thrown into the sea by a shipwrecked sailor, with no realistic expectation of its being found soon but a slim hope that perhaps someday, somehow, someone will know of the sender’s fate.
If direct visits among stars are unlikely, we must turn to the alternative for making contact: exchanging messages. Here, the news is a lot better. We already use a messenger—light or, more generally, electromagnetic waves—that moves through space at the fastest speed in the universe. Traveling at 300,000 kilometers per second, light reaches the nearest star in only four years and does so at a tiny fraction of the cost of sending material objects. These advantages are so clear and obvious that we assume they will occur to any other species of intelligent beings that develop technology.
However, we have access to a wide spectrum of electromagnetic radiation, ranging from the longest-wavelength radio waves to the shortest-wavelength gamma rays. Which would be the best for interstellar communication? It would not be smart to select a wavelength that is easily absorbed by interstellar gas and dust or one that is unlikely to penetrate the atmosphere of a planet like ours. Nor would we want to pick a wavelength that has lots of competition for attention in our neighborhood.
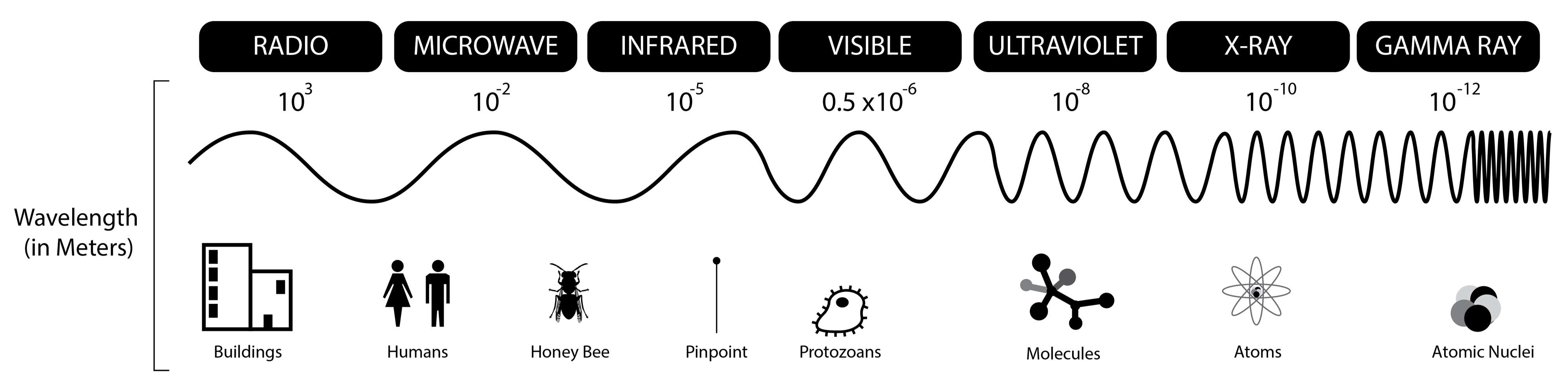
One final criterion makes the selection easier; we want the radiation to be inexpensive enough to produce in large quantities. When we consider all these requirements, radio waves turn out to be the best answer. Being the lowest-frequency (and lowest-energy) band of the spectrum, they are not very expensive to produce, and we already use them extensively for communications on Earth. They are not significantly absorbed by interstellar dust and gas. With some exceptions, they easily pass through Earth’s atmosphere and through the atmospheres of the other planets with which we are acquainted.
17.5.6 The Cosmic Haystack
Having made the decision that radio is the most likely means of communication among intelligent civilizations, we still have many questions and a daunting task ahead of us. Shall we send a message, or try to receive one? Obviously, if every civilization decides to receive only, then no one will be sending and everyone will be disappointed. On the other hand, it may be appropriate for us to begin by listening, since we are likely to be among the most primitive civilizations in the galaxy interested in exchanging messages.
We do not make this statement to insult the human species (of which, with certain exceptions, we are rather fond). Instead, we base it on the fact that humans have had the ability to receive (or send) a radio message across interstellar distances for only a few decades. Compared to the ages of the stars and the galaxy, this is a mere instant. If there are civilizations out there that are ahead of us in development by even a short time (in the cosmic sense), they are likely to have a technology head start of many, many years. In other words, we, who have just started, may well be the “youngest” species in the galaxy with this capability.
Just as the youngest members of a community are often told to be quiet and listen to their elders for a while before they say something foolish, so may we want to begin our exercise in extraterrestrial communication by listening. Even restricting our activities to listening, however, leaves us with an array of challenging questions. For example, if an extraterrestrial civilization’s signal is too weak to be detected by our present-day radio telescopes, we will not detect them. In addition, it would be very expensive for an extraterrestrial civilization to broadcast on a huge number of channels. Most likely, they select one or a few channels for their particular message. Communicating on a narrow band of channels also helps distinguish an artificial message from the radio static that comes from natural cosmic processes. But the radio band contains an astronomically large number of possible channels. How can we know in advance which one they have selected and how they have coded their message into the signal?
Because their success depends on either guessing right about many factors or searching through all the possibilities for each factor, some scientists have compared their quest to looking for a needle in a haystack. However, this has not stopped scientists from trying. Receivers are constantly improving, and the sensitivity of search for extraterrestrial intelligence (SETI) programs is advancing rapidly. Equally important, modern electronics and software allow simultaneous searches on millions of frequencies (channels). If we can thus cover a broad frequency range, the cosmic haystack problem of guessing the right frequency largely goes away. One powerful telescope array (funded with an initial contribution from Microsoft founder Paul Allen) built for SETI searches is the Allen Telescope in Northern California.
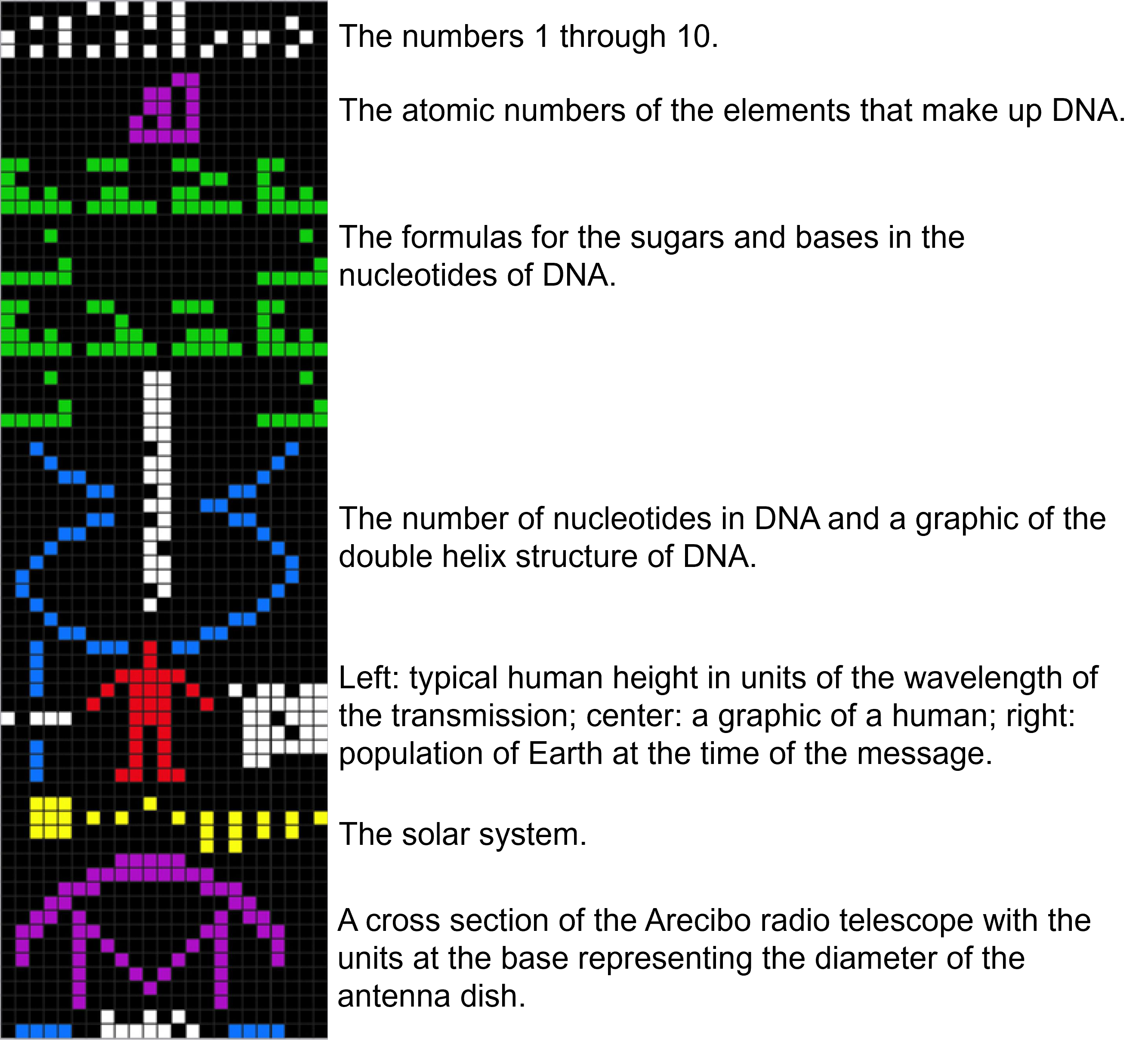
Other radio telescopes being used for such searches have included the giant Arecibo radio dish in Puerto Rico (which, sadly, collapsed due to storm damage in late 2020); the recently completed, and even larger, FAST dish in China; and the Green Bank Telescope in West Virginia, which is the largest steerable radio telescope in the world.
What kind of signals do we hope to pick up? We on Earth are inadvertently sending out a flood of radio signals, dominated by military radar systems. This is a kind of leakage signal, similar to the wasted light energy that is beamed upward by poorly designed streetlights and advertising signs. Could we detect a similar leakage of radio signals from another civilization? The answer is just barely, but only for the nearest stars. For the most part, therefore, current radio SETI searches are looking for beacons, assuming that civilizations might be intentionally drawing attention to themselves or perhaps sending a message to another world or outpost that lies in our direction. Our prospects for success depend on how often civilizations arise, how long they last, and how patient they are about broadcasting their locations to the cosmos.
17.5.7 The Fermi Paradox
If the Copernican principle is applied to life, then biology may be rather common among planets. Taken to its logical limit, the Copernican principle also suggests that intelligent life like us might be common. Intelligence like ours has some very special properties, including an ability to make progress through the application of technology. Organic life around other (older) stars may have started a billion years earlier than on Earth, so they may have had a lot more time to develop advanced technology, such as sending information, probes, or even life forms between stars.
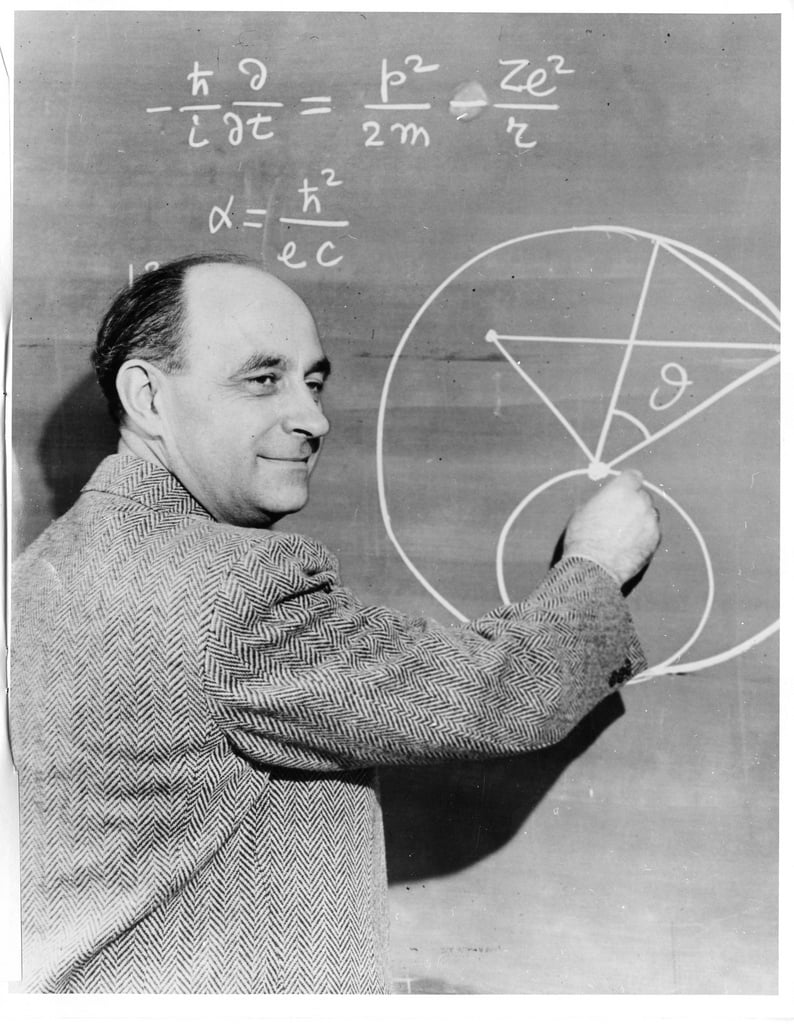
Faced with such a prospect, physicist Enrico Fermi asked a question several decades ago that is now called the Fermi paradox: “Where are they?” If life and intelligence are common and have such tremendous capacity for growth, why is there not a network of galactic civilizations whose presence extends even into a “latecomer” planetary system like ours?
Several solutions have been suggested to the Fermi paradox. Perhaps life is common, but intelligence (or at least technological civilization) is rare. Perhaps such a network will come about in the future but has not yet had the time to develop. Maybe there are invisible streams of data flowing past us all the time that we are not advanced enough or sensitive enough to detect. Maybe advanced species make it a practice not to interfere with immature, developing consciousnesses such as our own. Or perhaps civilizations that reach a certain level of technology then self-destruct, meaning there are no other civilizations now existing in our galaxy. We do not yet know whether any advanced life is out there, and if it is, we don’t know why we aren’t aware of it.
Summary
Our Solar System currently consists of the Sun, eight planets, five dwarf planets, nearly 200 known moons, and a host of smaller objects. The planets can be divided into two groups: the inner terrestrial planets and the outer giant planets. Smaller members of the Solar System include asteroids (including the dwarf planet Ceres), which are rocky and metallic objects found mostly between Mars and Jupiter; comets, which are made mostly of frozen gases and generally orbit far from the Sun; and countless smaller grains of cosmic dust. When a meteor survives its passage through our atmosphere and falls to Earth, we call it a meteorite.
The ages of the surfaces of objects in the Solar System can be estimated by counting craters; on a given world, a more heavily cratered region will generally be older than one that is less cratered. We can also use samples of rocks with radioactive elements in them to obtain the time since the layer in which the rock formed last solidified. The half-life of a radioactive element is the time it takes for half the sample to decay; we determine how many half-lives have passed by how much of a sample remains the radioactive element and how much has become the decay product. In this way, we have estimated the age of the Moon and Earth to be roughly 4.5 billion years.
Regularities among the planets have led astronomers to hypothesize that the Sun and the planets formed together in a giant, spinning cloud of gas and dust called the solar nebula. Astronomical observations show tantalizingly similar circumstellar disks around other stars. Within the solar nebula, material first coalesced into planetesimals; many of these gathered together to make the planets and moons. The remainder can still be seen as comets and asteroids. All planetary systems have probably formed in similar ways, but many exoplanet systems have evolved along quite different paths.
Life is made up of chemical combinations of elements made by stars. The Copernican principle, which suggests that there is nothing special about our place in the universe, implies that life’s development on Earth means that it should be able to develop in other places as well. The Fermi paradox asks why, if life is common, more advanced life forms have not contacted us.
The search for life beyond Earth offers several intriguing targets. Mars appears to have been more similar to Earth during its early history than it is now, with evidence for liquid water on its ancient surface and perhaps even now below ground. In the outer Solar System, the moons Europa and Enceladus likely host vast sub-ice oceans that may directly contact the underlying rocks—a good start in providing habitable conditions—while Titan offers a fascinating laboratory for understanding the sorts of organic chemistry that might ultimately provide materials for life. The last decade of research on exoplanets leads us to believe that there may be billions of habitable planets in the Milky Way galaxy. Study of these worlds offers the potential to find biomarkers indicating the presence of life.
Some astronomers are engaged in the search for extraterrestrial intelligent life (SETI). Because other planetary systems are so far away, traveling to the stars is either very slow or extremely expensive (in terms of energy required). Despite many UFO reports and tremendous media publicity, there is no evidence that any of these instances are related to extraterrestrial visits. Scientists have determined that the best way to communicate with any intelligent civilizations out there is by using electromagnetic waves, and radio waves seem best suited to the task. So far, they have only begun to comb the many different possible stars, frequencies, signal types, and other factors that make up what we call the cosmic haystack problem.
Take this quiz to check your comprehension of this chapter.
If you are using an offline version of this text, access the quiz for Chapter 17 via the QR code.
Text References
- Parts of this chapter are from OpenStax’s Astronomy 2e (2022). CC BY 4.0. Specifically, section 17.2 can be accessed for free at https://openstax.org/books/astronomy-2e/pages/7-1-overview-of-our-planetary-system, section 17.3 can be accessed for free at https://openstax.org/books/astronomy-2e/pages/7-2-composition-and-structure-of-planets, section 17.3.5 can be accessed for free at https://openstax.org/books/astronomy-2e/pages/7-3-dating-planetary-surfaces, section 17.4 can be accessed for free at https://openstax.org/books/astronomy-2e/pages/7-4-origin-of-the-solar-system, and section 17.5 can be accessed for free at https://openstax.org/books/astronomy-2e/pages/30-thinking-ahead.
- Chapter 17 Origin of Earth and the Solar System (CC BY 4.0) by Karla Panchuk was added from Earle, S. (2019). Physical geology, 2nd edition: BCcampus. https://opentextbc.ca/physicalgeology2ed/chapter/22-1-starting-with-a-big-bang
Figure References
Figure 17.1: The big bang. NASA/WMAP Science Team. 2006. Public domain. https://en.wikipedia.org/wiki/File:CMB_Timeline300_no_WMAP.jpg
Figure 17.2: Cosmic microwave background (CMB) map of the sky, a baby picture of the universe. NASA/WMAP Science Team. 2012. Public domain. https://commons.wikimedia.org/wiki/File:Ilc_9yr_moll4096.png
Figure 17.3: Doppler effect. Charly Whisky. 2007. CC BY-SA 3.0. https://commons.wikimedia.org/wiki/File%3ADopplerfrequenz.gif
Figure 17.4: Red shift in light from the supercluster BAS11 compared to the Sun’s light. Kindred Grey. 2022. CC BY 4.0. Includes Duck by parkjisun from Noun Project (Noun Project license).
Figure 17.5: Astronauts on the Moon. NASA Johnson Space Center; Restored by Bammesk. 1971. Public domain. https://en.wikipedia.org/wiki/File:AS15-88-11866_-_Apollo_15_flag,_rover,_LM,_Irwin_-_restoration1.jpg
Figure 17.6: Orbits of the planets. Arabik4892. 2022. CC BY-SA 4.0. https://commons.wikimedia.org/wiki/File:Planet_Orbits.jpg
Figure 17.7: Surface of Mercury. NASA/Johns Hopkins University Applied Physics Laboratory/Carnegie Institution of Washington. 2009. Public domain. https://commons.wikimedia.org/wiki/File:Mercury_Double-Ring_Impact_Basin.png
Figure 17.8: Mars Express orbiter view of Olympus Mons with its summit caldera, escarpment, and aureole. ESA/DLR/FUBerlin/AndreaLuck. March 2023. CCY BY. https://en.wikipedia.org/wiki/Olympus_Mons#/media/File:Olympus_Mons_-_ESA_Mars_Express_-_Flickr_-_Andrea_Luck.png
Figure 17.9: The four giant planets. Solar System Exploration, NASA. 2008. Public domain. https://commons.wikimedia.org/wiki/File:Gas_planet_size_comparisons.jpg
Figure 17.10: This intriguing image from the New Horizons spacecraft, taken when it flew by the dwarf planet Pluto in July 2015, shows some of its complex surface features. NASA / Johns Hopkins University Applied Physics Laboratory/Southwest Research Institute. 2015. Public domain. https://en.wikipedia.org/wiki/File:Pluto-01_Stern_03_Pluto_Color_TXT.jpg
Figure 17.11: Saturn and its A, B, and C rings in visible and (inset) infrared light. NASA/JPL-Caltech/Space Science Institute/G. Ugarkovic (ISS), NASA/JPL-Caltech/University of Arizona/CNRS/LPG-Nantes (VIMS). 2019. Public domain. https://commons.wikimedia.org/wiki/File:PIA23170-Saturn-Rings-IR-Map-20190613.jpg
Figure 17.12: Asteroid Eros. NASA/JPL/JHUAPL. 2000. Public domain. https://commons.wikimedia.org/wiki/File:Eros_-_PIA02923_(color).jpg
Figure 17.13: Comet Churyumov-Gerasimenko (67P). ESA/Rosetta/MPS for OSIRIS Team MPS/UPD/LAM/IAA/SSO/INTA/UPM/DASP/IDA. 2014. CC BY-SA 4.0. https://commons.wikimedia.org/wiki/File:Comet_67P_True_color.jpg
Figure 17.14: Generalized internal structure of terrestrial planets. Wikimedia Commons. Retrieved October 2024. Public domain. https://commons.wikimedia.org/wiki/File:Terrestial_Planets_internal_en.jpg
Figure 17.15: Jupiter with its moon Europa on the left. NASA, ESA, STScI, A. Simon (Goddard Space Flight Center), and M.H. Wong (University of California, Berkeley) and the OPAL team. 2020. Public domain. https://commons.wikimedia.org/wiki/File:Jupiter_and_Europa_2020.tiff
Figure 17.16: Jupiter’s moon Ganymede. NOAA. 2009. Public domain. https://commons.wikimedia.org/wiki/File:Moon_Ganymede_by_NOAA.jpg
Figure 17.17: The present-day atmosphere of Venus. J.G. O’Rourke, C.F. Wilson, and M.E. Borrelli, et al. February 2023. CC BY-SA. https://en.wikipedia.org/wiki/Atmosphere_of_Venus#/media/File:11214_2023_956_Fig6_HTML.webp
Figure 17.18: Our cratered Moon. NASA/Goddard/Arizona State University. 2011. Public domain. https://www.nasa.gov/mission_pages/LRO/news/lro-farside.html
Figure 17.19: Radioactive decay. Andrew Fraknoi, David Morrison, and Sidney Wolff. 2015. CC BY 4.0. https://en.wikipedia.org/wiki/File:OSC_Astro_07_03_Decay_(1).jpg
Figure 17.20: Sample from NASA’s lunar surface collection at Johnson Space Center’s vault in Houston, Texas. Shannon Moore. 8 August 2011. CC BY. https://commons.wikimedia.org/wiki/File:Moon_rock_4,_JSC.jpg
Figure 17.21: NASA artist’s conception of various planet formation processes, including exocomets and other planetesimals, around Beta Pictoris, a very young type-AV star. NASA/FUSE/Lynette Cook. 2007. Public domain. https://commons.wikimedia.org/wiki/File:NASA-ExocometsAroundBetaPictoris-ArtistView.jpg
Figure 17.22: Atlas of planetary nurseries. Credit: modification of work by NASA ESA/Hubble, L. Ricci (ESO). 2009. CC BY 4.0. https://esahubble.org/copyright/
Figure 17.23: This illustration demonstrates how a massive star (at least eight times bigger than our sun) fuses heavier and heavier elements until exploding as a supernova and spreading those elements throughout space. “Massive Stars: Engines of Creation.” NASA, ESA, and L. Hustak. 2019. Public domain. https://hubblesite.org/contents/media/images/4501-Image.html
Figure 17.24: NASA’s Mars Reconnaissance Orbiter used its Context Camera to capture this image of Bosporos Planum, a location on Mars. NASA/JPL-Caltech/MSSS. 2022. Public domain. https://www.nasa.gov/solar-system/nasas-mro-finds-water-flowed-on-mars-longer-than-previously-thought
Figure 17.25: The hole that NASA’s Curiosity Mars rover drilled into a mudstone. NASA/JPL-Caltech/MSSS. 2013. Public domain. https://science.nasa.gov/resource/view-into-john-klein-drill-hole-in-martian-mudstone
Figure 17.26: Artist’s concept of Jupiter’s moon Europa. NASA/JPL-Caltech. 2013. Public domain. https://www.nasa.gov/image-article/taste-of-ocean-europas-surface
Figure 17.27: Images of Saturn’s moon Titan taken in 2005 by the Huygens probe at four different altitudes. ESA/NASA/JPL/University of Arizona. 2005. Public domain. https://www.nasa.gov/image-article/ten-years-ago-huygens-probe-lands-surface-of-titan
Figure 17.28: Diagram explaining the habitable zone. Chester Harman. 2017. CC BY-SA. https://commons.wikimedia.org/wiki/File:Diagram_of_different_habitable_zone_regions_by_Chester_Harman.jpg
Figure 17.29: The Golden Record cover shown with its extraterrestrial instructions. NASA/JPL. 1977. Public Domain. https://voyager.jpl.nasa.gov/golden-record/golden-record-cover
Figure 17.30: The electromagnetic (EM) spectrum. Jonathan S Urie. 2013. CC BY-SA. https://commons.wikimedia.org/wiki/File:BW_EM_spectrum.png
Figure 17.31: A graphical representation of the Arecibo message. Laura Neser, adapted from Arne Nordmann. 2005. CC BY-SA. https://commons.wikimedia.org/wiki/File:Arecibo_message.svg
Figure 17.32: Enrico Fermi. Smithsonian Institution Archives. 1950. Public domain. https://commons.wikimedia.org/wiki/File:Enrico_Fermi_at_the_blackboard.jpg
Figure Descriptions
Figure 17.1: Big Bang Expansion began 13.77 billion years ago. From inception towards present: quantum fluctuations, inflation, afterglow light pattern (375,000 years), dark ages, first stars (400 million years), development of galaxies and planets, dark energy accelerated expansion.
Figure 17.2: An oval-shaped map of cosmic background radiation from when the universe was around 375,000 years old. The colors are artificial and show tiny temperature variations: tiny red patches have the highest temperature and the more widespread blue patches have the lowest temperature.
Figure 17.3: The ripples made in the direction the car is moving are closer together than the ripples behind the car.
Figure 17.4: Two spectrums of light stacked on top of each other: Sun on top, BAS11 on bottom. Arrows from the Sun toward BAS11 convey a slight shift of wavelength toward the longer wavelength colors. A duck swimming toward the left on rippled water is directly above the diagram, with shorter wavelength ripples in front of the duck and longer wavelength ripples behind the duck.
Figure 17.5: Photograph of Astronauts on the Moon. At center is the landing module, and to the right is the Lunar rover used by the Astronauts to travel large distances from the landing site. At left an Astronaut salutes the American flag placed near the lander. Scattered throughout the foreground are footprints in the Lunar soil.
Figure 17.6: Diagram of solar system objects orbiting the Sun. The objects plotted in the diagram moving outward from the Sun are Mercury, Venus, Earth, Mars, Asteroid belt, Jupiter, Saturn, Uranus, Neptune, and Pluto. All of the objects orbit the Sun in roughly the same plane with the exception of Pluto whose orbit is tilted with respect to the ecliptic.
Figure 17.7: Overhead view of the gray cratered surface of Mercury. Large craters, with many overlapping one upon the other, cover the surface of this 400 km wide scene.
Figure 17.8: Satellite image showing a reddish tan expanse with a large circular gently sloping volcano emerging from the surrounding lanscape.
Figure 17.9: Diagram of the four giant planets and Earth shown to scale according to size (not according to distance). Arranged from left to right are Jupiter, Saturn, Uranus, and Neptune, with Earth below the giant planets.
Figure 17.10: Enhanced-color image of the surface of Pluto. In this photograph, the smooth, white Sputnik plains are seen covering the center right of the image. Rugged, heavily cratered maroon terrain covers the lower center and left.
Figure 17.11: Image taken almost directly over one of Saturn’s poles; Saturn’s rings are seen nearly face-on, completely encircling the planet. Sunlight arrives from lower left as the rings cast a thin shadow on Saturn’s cloud tops, while Saturn itself casts a shadow on the rings on the left. A rectangular false-color image at the right shows spectral mapping of Saturn’s A, B and C rings: blue-green areas are the regions with the purest water ice and/or largest grain size (primarily the A and B rings), while the reddish color indicates increasing amounts of non-icy material and/or smaller grain sizes (primarily in the C ring and Cassini Division).
Figure 17.12: Gray asteroid that is very irregular in shape, in this case similar to a potato. The surface is pock-marked with many craters, including a 5.3- kilometer diameter crater at the top.
Figure 17.13: Gray comet that is very irregular in shape, in this case similar to a dumbbell. The surface appears powdery and pock-marked.
Figure 17.14: Mercury is a small planet and has a large core and thin mantle. Venus is a large planet and has a larger core and smaller mantle. Earth is similar to Venus, except it has a solid inner core and liquid outer core. Earth’s Moon is small and might have a core, but is mostly mantle. Mars is a medium sized planet and has a smaller core and larger mantle. All of the planets have a thin crust.
Figure 17.15: Image of planet Jupiter with a tiny gray moon to its left. The planet Jupiter has multicolored white, tan, and red bands along various latitudes and there is a large red spot visible just below its equator.
Figure 17.16: Photo of a moon with a brownish gray surface and small craters scattered across the entire surface. There are a few bright white spots where recent impacts have uncovered fresh ice from underneath.
Figure 17.17: Graph with temperature on the horizontal axis increasing from 100 kelvins on the left to 800 kelvins on the right. The vertical axes have altitude increasing upward and pressure decreasing upward. There is a bold black line that starts at 0 km altitude and 750 kelvins; as it goes upward it goes to the left toward lower temperatures at higher altitude, nearly flattening into a vertical line around 90 km altitude.
Figure 17.18: View of the light gray cratered far side surface of our Moon. Craters of many sizes cover the surface, many of them overlapping. There are a few darker gray areas on the Moon in the upper left and lower portion of the image.
Figure 17.19: Graph Illustrating the Concept of Radioactive Decay. The vertical scale is labeled “Fraction of Original Sample Remaining”, and increases from 0 to 1.0 in increments of 0.1. The horizontal scale is labeled “Number of Half- lives”, and increases to the right from 0 to 5 in increments of 1. A curve is drawn from (0, 1.0) at upper left down to (5, 0) at lower right. A dashed line is drawn vertically upward from 1 to intersect the curve at 0.5 on the vertical scale. At this point on the curve 1/2 of the original material remains. Next, another dashed line is drawn vertically upward from 2 to intersect the curve at 0.25, where 1/4 of the original sample remains. Another dashed line is drawn upward from 3 to intersect the curve at 0.125, where 1/8 of the sample remains. Again, a dashed line is drawn upward from 4 to intersect the curve at 0.06, where 1/16 of the sample remains. Finally, the dashed line from 5 intersects the curve at 0.03, where 1/32 of the original sample remains. Above the curve are drawn six “blobs” of material, one for each data point. The blob is pink at the top of the curve representing the full radioactive sample. The blob at (1, 0.5) is about 1/2 pink and 1/2 grey representing the fact that 1/2 of the original sample remains. This illustration continues for the remaining data points so that by (5, 0.03) the blob is nearly all grey indicating that only 1/32 of the original sample remains.
Figure 17.20: Photo of a gray rock with black specks throughout it. The rock is sitting on a white sheet of paper with a metal ruler in the foreground and a black die next to it. There is a label that says 15555 in front of the rock.
Figure 17.21: Artist’s conception of the view toward the young star Beta Pictoris from the outer edge of its disk. The star is surrounded by a disk of dust, gas, and rocks. A terrestrial planet gaining mass by collision with an asteroid is shown just to the right of center. Two inset panels show two possible outcomes for mature terrestrial planets around the star. The top one is a water-rich planet similar to the Earth; the bottom one is a carbon-rich planet, with a smoggy, methane-rich atmosphere similar to that of Titan, a moon of Saturn.
Figure 17.22: A Photographic Atlas of Planetary Nurseries in the Orion Nebula. These Hubble Space Telescope images show embedded circumstellar disks orbiting very young stars. Each is seen from a different angle. Some are energized to glow brightly by the light of a nearby star, while others are dark and seen in silhouette against the bright glowing gas of the Orion nebula.
Figure 17.23: Infographic showing a purple sphere with a cut-out to show the core, labeled main sequence star: the star is fueled by hydrogen-to-helium fusion in its core; stage lasts 7 million years. A dotted line connects it to a larger pink sphere with a cut-out to show the core, labeled development of multi-shelled core: after hydrogen is exhausted in the core, other elements begin to fuse; existing elements float above the denser center and form a multi-layered shell. The multi-shell core is zoomed to a larger size below and divided into four progressive stages. The first is a simple two-layered core labeled helium-to-carbon fusion; 500,000 years. To the right of that, the multi-shell core is made of three layers and labeled carbon-to-oxygen fusion; 600 years. To the right of that, the multi-shell core is made of four layers and labeled oxygen-to-silicon fusion; 6 months. To the right of that, the multi-shell core is made of five layers and labeled silicon-to-iron fusion; 1 day. Lastly, there is a purplish blob of gas following the silicon-to- iron fusion core, labeled Supernova: the iron core cannot produce energy to balance the weight of the layered gas above. In less than one second, the core collapses and the upper layers rebound outward in a dramatic explosion, populating the universe with elements, the building blocks of creation; 0.01 seconds.
Figure 17.24: Black and white aerial photograph of a generally flat area. There are channels resembling the shapes of rivers with white specks seen throughout the channels. Craters of various sizes dot the surface as well.
Figure 17.25: Closeup photograph of a hole drilled into tan fine-grained rock. There’s a black scale bar labeled 2 mm to the right of the hole, showing the hole is approximately 16 mm in diameter.
Figure 17.26: Illustration showing a cross section of a moon in the foreground that has a brown crust with a thick layer of ice directly below the outer crust and a deep blue ocean beneath the ice. Multiple geysers branch vertically through the ice from the top of the ocean, causing eruptions on the surface of the moon. In the background is the planet Jupiter and a small yellow moon.
Figure 17.27: Four aerial photos getting progressively closer to the surface showing a sepia-orangeish colored landscape. The first photo shows a general orangeish haze, the second photo shows a wide landscape with branching patterns, the third photo shows the branching patterns from a lower altitude, and the final photo is closer to the ground, showing the orangeish landscape sprawling away from the camera.
Figure 17.28: A graph that has temperature in K increasing upwards on the vertical axis and starlight on planet relative to sunlight on Earth increasing toward the left on the horizontal axis. A large shaded zone covers most of the graph labeled optimistic habitable zone. Various exoplanets are shown within the optimistic habitable zone along with Earth, Venus, and Mars, with Earth and Mars within the habitable zone and Venus outside and to the left of the habitable zone.
Figure 17.29: A photo of a golden record on the left that has various etchings visible on the surface. Including: a basic birds-eye view drawing of a music record and stylus, a side view of the flat record and stylus, graphs describing the construction of pictures from the recorded signals, a drawing of an entire picture raster, showing that there are 512 vertical lines in a complete picture, a rectangle with a circle in the center, a replica of the first picture of a birds-eye view of a record. A schematic diagram on the right shows etchings in black and white.
Figure 17.30: A black sinusoidal wave goes from the left to the right of the diagram with longer wavelengths to the left, getting progressively shorter and shorter to the right. The types of waves are labeled based on their wavelengths: from longest to shortest they are radio, microwave, infrared, visible, ultraviolet, x-ray, and gamma ray. Common things on Earth are beneath the waves for scale: from left to right they are buildings, humans, honey bee, pinpoint, protozoans, molecules, atoms, and atomic nuclei.
Figure 17.31: A colored graphical representation that consists of seven parts that encode the following from the top down in the image: the numbers one to ten in white; the atomic numbers of the elements hydrogen, carbon, nitrogen, oxygen, and phosphorus, which make up DNA in purple; the formulas for the chemical compounds that make up the nucleotides of DNA in green; the estimated number of DNA nucleotides in the human genome, and a graphic of the double helix structure of DNA in white and blue, respectively; the physical height of an average man in blue and white, a graphic figure of a human being in red, and the human population of Earth which was about 4 billion at the time in white; a graphic of the Solar System in yellow; and a graphic of the Arecibo radio telescope and the physical diameter of the transmitting antenna dish in purple, white, and blue.
Figure 17.32: Black and white photograph of a white man wearing a chevron-textured suit writing on a chalkboard with his face turned back away from the board. There are various equations and geometric shapes drawn on the chalkboard.
All of space and time and their contents, including planets, stars, galaxies, and all other forms of matter and energy.
A process inside stars in which smaller atoms combine and form larger atoms.
Large explosion when the largest stars end fusion; responsible for the formation of heavy elements in the universe, like gold and uranium.
Any planet beyond our Solar System.
The theory that the universe started with a expansive explosion, followed by the creation of elements (mostly hydrogen) and the formation of galaxies.
The distance that light can travel through space in a year. One light year is 9.4607 × 10^12 km.
Radiation left over from the early stage in the development of the universe, when protons and neutrons were recombining to form atoms.
A gravitationally bound system of stars and interstellar matter.
Increase in the wavelength of light resulting from the light source moving away from the observer.
A change in wavelength and frequency of a wave due to its source's movement relative to the observer of a wave.
The generic term for a group of planets and other bodies circling a star.
To move in a circular or curving course or orbit. Not to be confused with rotate (to spin on an axis).
A small planetary-mass object that is in direct orbit of the Sun; smaller than any of the eight classical planets, but still a world in its own right.
A circumstellar disc in the outer Solar System, extending from the orbit of Neptune at 30 astronomical units (AU) to approximately 50 AU from the Sun.
A spherical layer of icy objects surrounding the Sun; likely occupies space at a distance between about 2,000 and 100,000 AU from the Sun.
The branch of science that deals with celestial objects, space, and the physical universe as a whole.
In planetary science, the process of separating the different components within a planetary body as a consequence of their physical or chemical behavior (e.g., density and chemical affinities).
A reddish-colored cyclonic storm that circulates counterclockwise in the southern hemisphere of Jupiter's atmosphere. Continuously observed since the seventeenth century, the storm is so large that it could fit multiple Earths within its boundaries.
The average distance from the Earth to the Sun.
A penetrating form of electromagnetic radiation arising from the radioactive decay of atomic nuclei. Its electronic waves have the shortest wavelengths, typically shorter than those of X-rays.
Rotating, flattened disk of gas and dust from which the Solar System originated.
The study of the details of light, which can tell you the chemical makeup of light and even the movement of a light source.
A body that could or did come together with many others under gravitation to form a planet.
The scientific study of life in the universe, including its origins, evolution, and potential existence beyond Earth.
A detectable substance or characteristic in an astronomical body's atmosphere or surface that indicates the potential presence of life.
A molecule that contains carbon atoms bonded together, often with hydrogen, oxygen, nitrogen, and other elements, forming the basis of life on Earth.
The branch of science that explores the chemical processes and substances that occur within living organisms.
The Copernican principle posits that the Earth is not in a central, specially favored position in the universe.
Complex organic compounds formed by the irradiation of simple carbon-containing molecules, often found on the surfaces of icy bodies in the outer Solar System.
The region around a star where conditions are suitable for liquid water to exist on a planet's surface, potentially allowing for life as we know it.
Occurring or situated between stars.
The constant velocity at which light travels in a vacuum, approximately 299,792 kilometers per second (186,282 miles per second).
Waves of electric and magnetic fields that propagate through space at the speed of light, encompassing a broad spectrum that includes radio waves, microwaves, infrared, visible light, ultraviolet, X-rays, and gamma rays.
The energy emitted and propagated through space in the form of electromagnetic waves.
SETI, or the Search for Extraterrestrial Intelligence, is a scientific effort aimed at detecting signals or evidence of intelligent life beyond Earth.
The Fermi paradox highlights the contradiction between the high probability of the existence of extraterrestrial civilizations and the lack of evidence or contact with such civilizations.
The branch of chemistry that studies the structure, properties, composition, reactions, and synthesis of carbon-containing compounds.