9 Crustal Deformation and Earthquakes
Learning Objectives
By the end of this chapter, students should be able to:
- Differentiate between stress and strain.
- Identify the three major types of stress.
- Differentiate between brittle, ductile, and elastic deformation.
- Describe the geological map symbol used for strike and dip of strata.
- Name and describe different fold types.
- Differentiate the three major fault types, and describe their associated movements.
- Explain how elastic rebound relates to earthquakes.
- Describe different seismic wave types and how they are measured.
- Explain how humans can induce seismicity.
- Describe how seismographs work to record earthquake waves.
- Locate the epicenter of an earthquake from seismograph records.
- Explain the difference between earthquake magnitude and intensity.
- List earthquake factors that determine ground shaking and destruction.
- Identify secondary earthquake hazards.
- Describe notable historical earthquakes.
Crustal deformation occurs when applied forces exceed the internal strength of rocks, physically changing their shapes. These forces are called stress, and the physical changes they create are called strain. Forces involved in tectonic processes, as well as gravity and igneous pluton emplacement, produce strains in rocks that include folds, fractures, and faults. When rock experiences large amounts of shear stress and breaks with rapid, brittle deformation, energy is released in the form of seismic waves, commonly known as an earthquake.
9.1 Stress and Strain
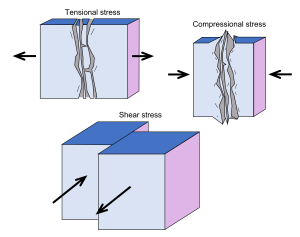
Stress is the force exerted per unit area, and strain is the physical change that results in response to that force. When applied stress is greater than the internal strength of rock, strain results in the form of deformation of the rock. Strain in rocks can be represented as a change in rock volume and/or rock shape, as well as fracturing of the rock. There are three types of stress: tensional, compressional, and shear. Tensional stress involves forces pulling in opposite directions, which results in strain that stretches and thins rock. Compressional stress involves forces pushing together, and compressional strain shows up as rock folding and thickening. Shear stress involves transverse forces; the strain shows up as opposing blocks or regions of material moving past each other.
Type of stress | Associated plate boundary type (see Chapter 2) | Resulting strain | Associated fault and offset types |
---|---|---|---|
Tensional | Divergent | Stretching and thinning | Normal |
Compressional | Convergent | Shortening and thickening | Reverse |
Shear | Transform | Tearing | Strike-slip |
Table 9.1: Types of stress and resulting strain.
Take this quiz to check your comprehension of this section.
If you are using an offline version of this text, access the quiz for Section 9.1 via the QR code.
9.2 Deformation
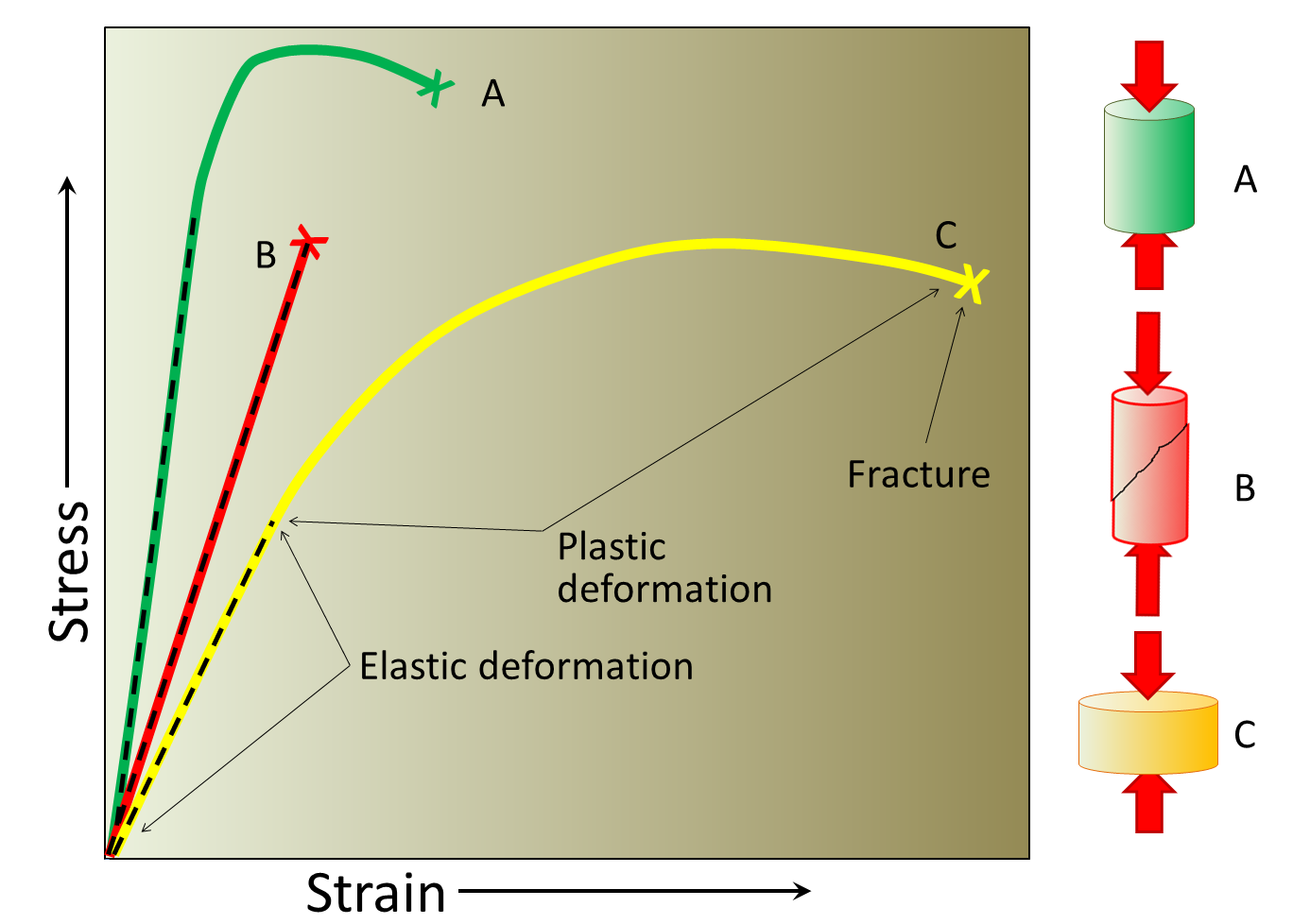
When rocks are stressed, the resulting strain can be elastic, ductile, or brittle. This change is generally called deformation. Elastic deformation is strain that is reversible after a stress is released. For example, when you stretch a rubber band, it elastically returns to its original shape after you release it. Ductile deformation occurs when enough stress is applied to a material that the changes in its shape are permanent, and the material is no longer able to revert to its original shape. For example, if you bend a metal bar too far, it can be permanently bent out of shape. The point at which elastic deformation is surpassed and strain becomes permanent is called the yield point. In Figure 9.2, the yield point is where the line transitions from elastic deformation to ductile deformation (the end of the dashed line). Brittle deformation is another critical point of no return, when rock integrity fails and the rock fractures under increasing stress.
The type of deformation a rock undergoes depends on pore pressure, strain rate, rock strength, temperature, stress intensity, time, and confining pressure. Pore pressure is exerted on the rock by fluids in the open spaces or pores embedded within rock or sediment. Strain rate measures how quickly a material is deformed. For example, applying stress slowly makes it is easier to bend a piece of wood without breaking it. Rock strength measures how easily a rock deforms under stress. Shale has low strength, and granite has high strength. Removing heat or decreasing temperature makes materials more rigid and susceptible to brittle deformation. On the other hand, heating materials make them more ductile and less brittle. Heated glass can be bent and stretched.
Factor | Strain response |
---|---|
Increase temperature | More ductile |
Increase strain rate | More brittle |
Increase rock strength | More brittle |
Table 9.2: Relationship between factors operating on rock and the resulting strains.
Take this quiz to check your comprehension of this section.
If you are using an offline version of this text, access the quiz for Section 9.2 via the QR code.
9.3 Geological Maps
Geologic maps are two-dimensional (2-D) representations of geologic formations and structures at the Earth’s surface, including formations, faults, folds, inclined strata, and rock types. Formations are recognizable rock units. Geologists use geologic maps to represent where geologic formations, faults, folds, and inclined rock units are. Each formation on the map is indicated by a color and a label. For examples of geologic maps, see the Utah Geological Survey (UGS) geologic map viewer.
Formation labels include symbols that follow a specific protocol. The first one or more letters are uppercase and represent the geologic time period of the formation. More than one uppercase letter indicates the formation is associated with multiple time periods. The following lowercase letters represent the formation name, abbreviated rock description, or both.
9.3.1 Cross Sections
Cross sections are subsurface interpretations made from surface and subsurface measurements. Maps display geology in the horizontal plane, while cross sections show subsurface geology in the vertical plane. For more information on cross sections, check out the AAPG wiki.
9.3.2 Strike and Dip
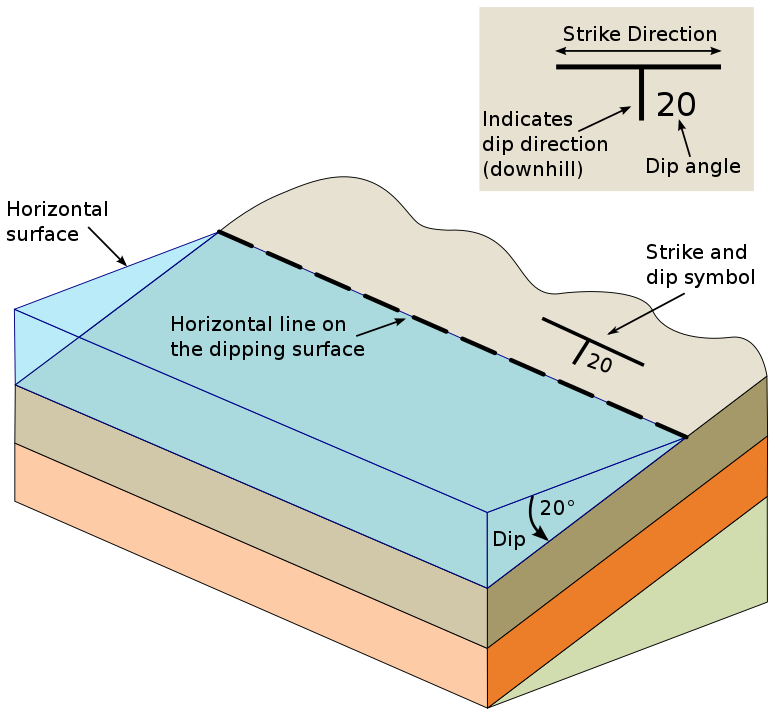
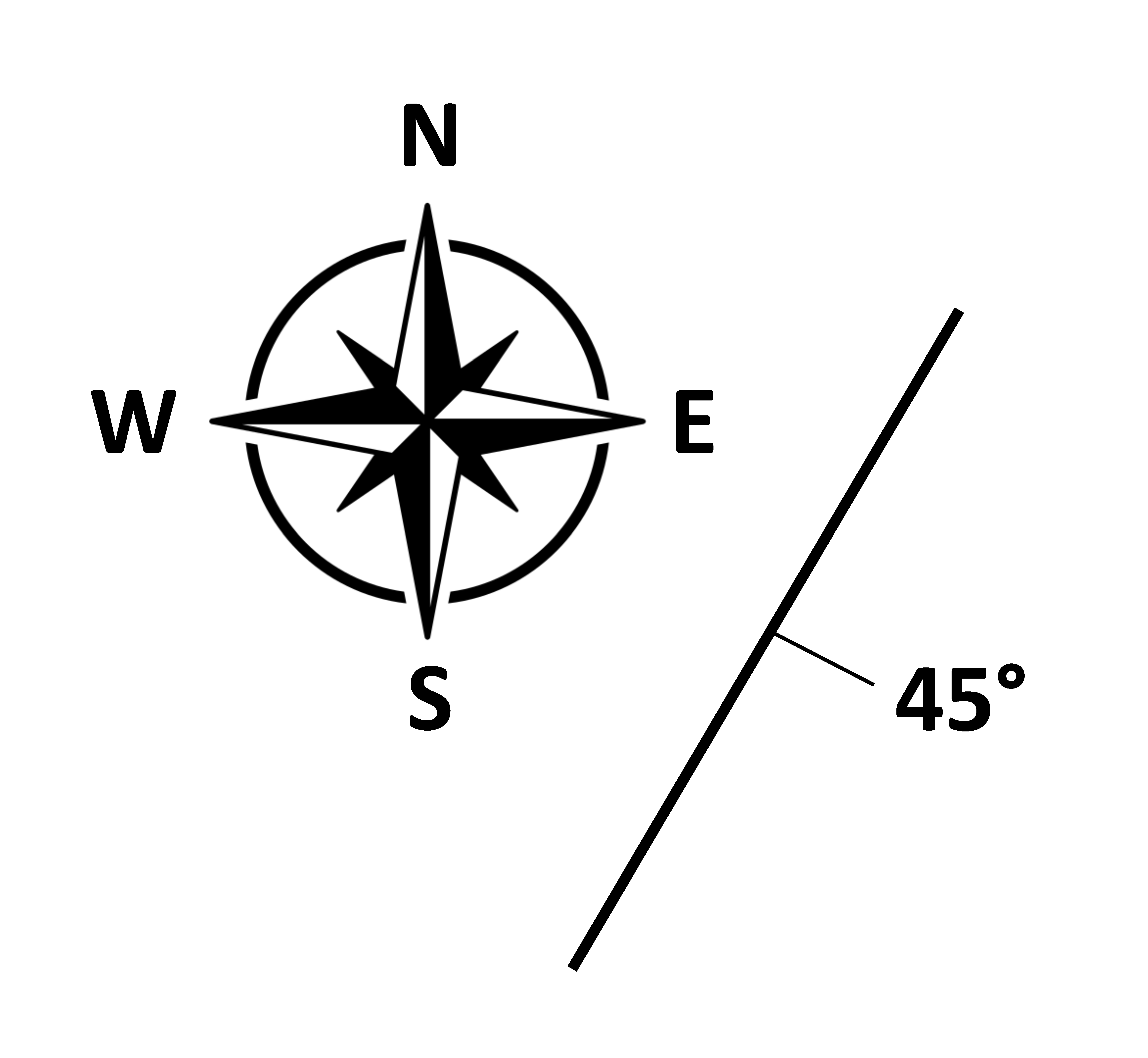
Geologists use a special symbol called strike and dip to represent inclined beds. Strike and dip map symbols look like the capital letter T, with a short trunk and extra-wide top line. The short trunk represents the dip, and the top line represents the strike. Dip is the angle that a bed plunges into the Earth from the horizontal. A number next to the symbol represents dip angle. One way to visualize the strike is to think about a line made by standing water on the inclined layer. That line is horizontal and lies on a compass direction that has some angle with respect to true north or south (see Figure 9.3). The strike angle is measured by a special compass—e.g., N 30° E (read north 30 degrees east) means the horizontal line points northeast at an angle of 30° from true north. The strike and dip symbol is drawn on the map at the strike angle with respect to true north on the map. The dip of the inclined layer represents the angle down to the layer from horizontal; in the figure, this is 45° SE (read dipping 45 degrees to the southeast). The direction of dip would be the direction a ball would roll if set on the layer and released. A horizontal rock bed has a dip of 0°, and a vertical bed has a dip of 90°. Strike and dip considered together are called rock attitude.
This video illustrates geologic structures and associated map symbols.
Video 9.1: Folds, dip, and strike
If you are using an offline version of this text, access this YouTube video via the QR code.
Take this quiz to check your comprehension of this section.
If you are using an offline version of this text, access the quiz for Section 9.3 via the QR code.
9.4 Folds
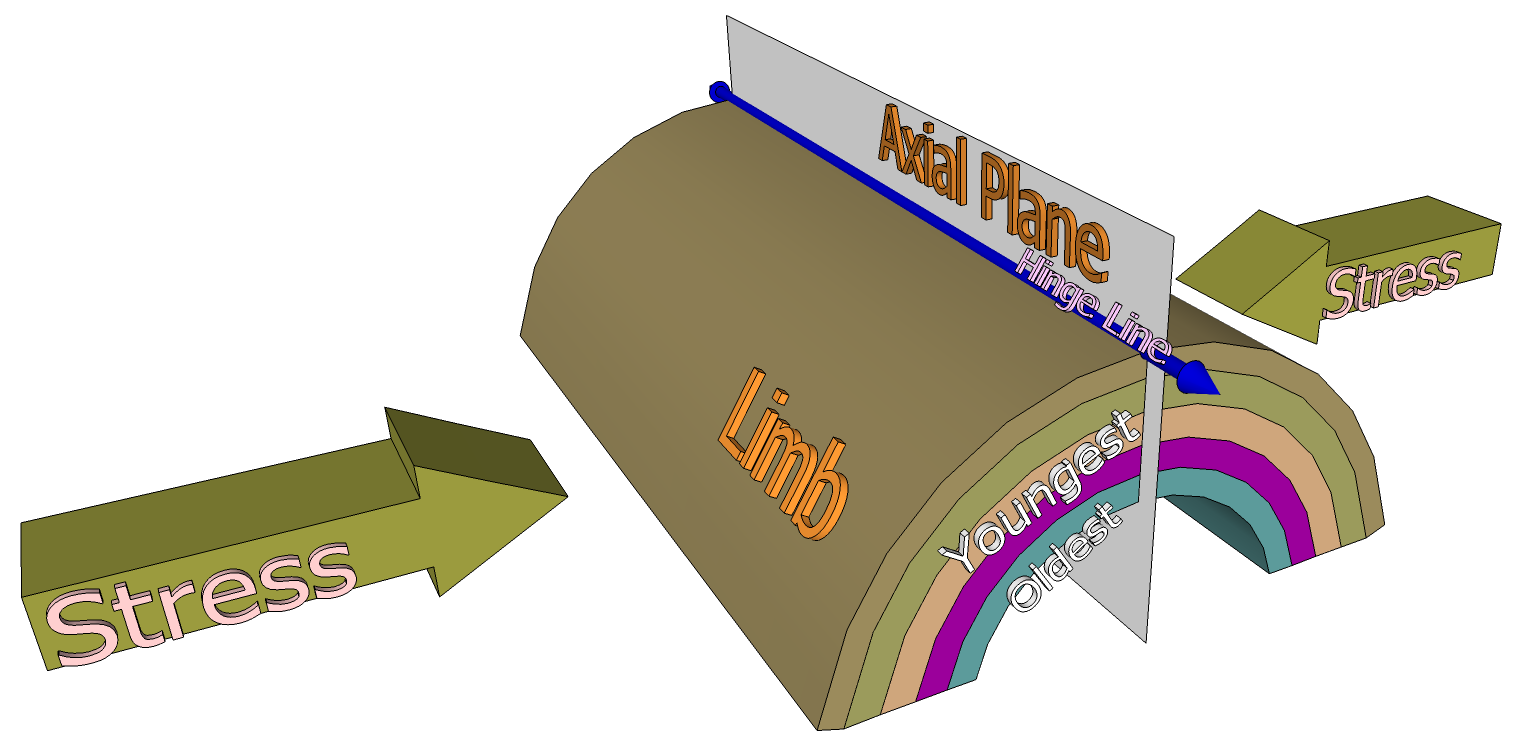
Geologic folds are layers of rock that are curved or bent by ductile deformation. Folds are most commonly formed by compressional forces at depth, where hotter temperatures and higher confining pressures allow ductile deformation to occur.
Folds are described by the orientation of their axes, axial planes, and limbs. The plane that splits the fold into two halves is known as the axial plane. The fold axis is the line along which the bending occurs and is where the axial plane intersects the folded strata. The hinge line follows the line of greatest bend in a fold. The two sides of the fold are the fold limbs.
Symmetrical folds have a vertical axial plane, and limbs have equal but opposite dips. Asymmetrical folds have dipping, nonvertical axial planes where the limbs dip at different angles. Overturned folds have steeply dipping axial planes, and the limbs dip in the same direction but usually at different dip angles. Recumbent folds have horizontal or nearly horizontal axial planes. When the axis of the fold plunges into the ground, the fold is called a plunging fold. Folds are classified into five categories: anticline, syncline, monocline, dome, and basin.
9.4.1 Anticline
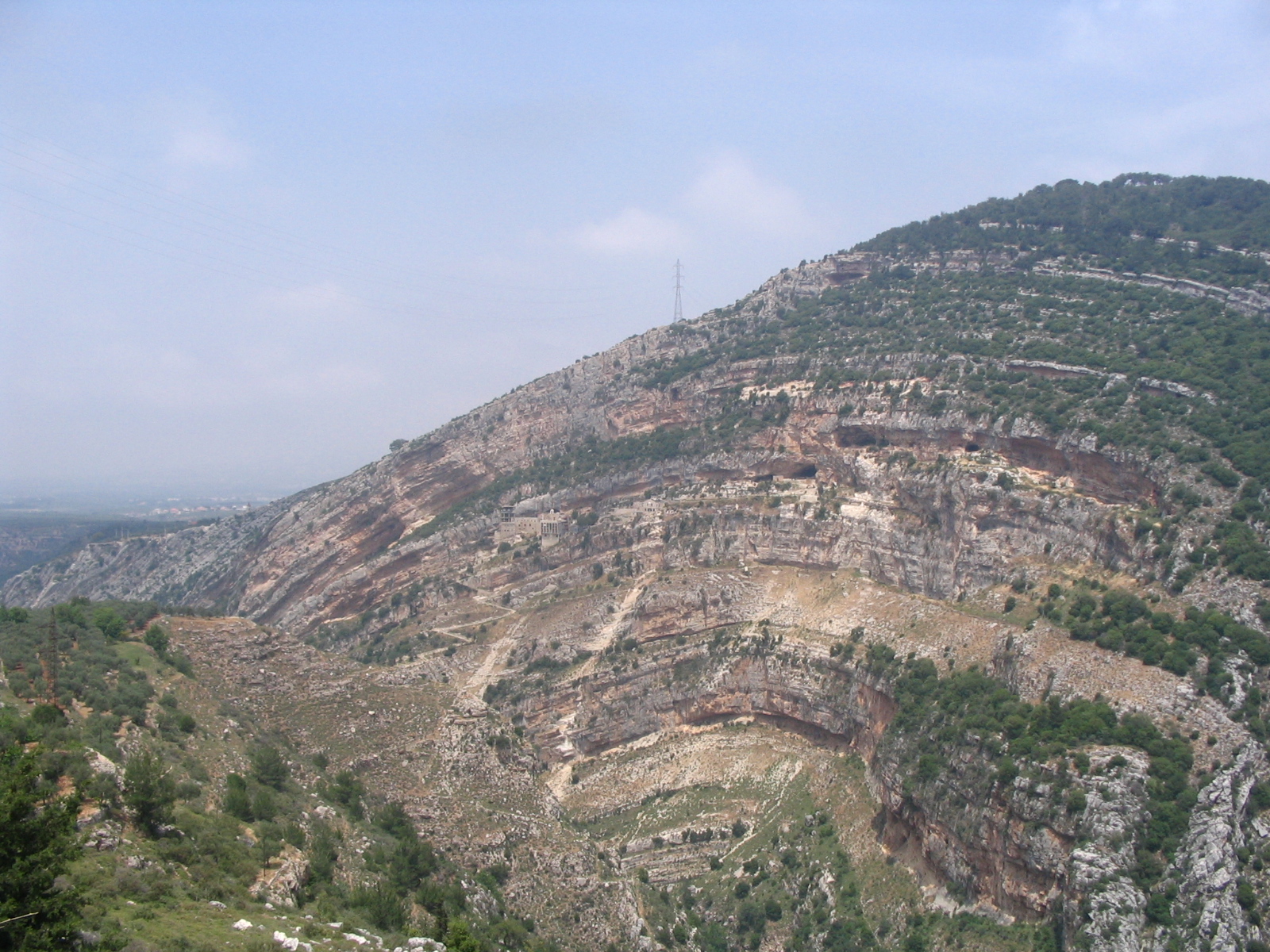
Anticlines are archlike, or A-shaped, folds that are convex-upward in shape. They have downward curving limbs and beds that dip down and away from the central fold axis. In anticlines, the oldest rock strata are in the center of the fold, along the axis, and the younger beds are on the outside. Since geologic maps show the intersection of surface topography with underlying geologic structures, an anticline on a geologic map can be identified by both the attitude of the strata forming the fold and the older age of the rocks inside the fold. An antiform has the same shape as an anticline, but the relative ages of the beds in the fold cannot be determined. Oil geologists are interested in anticlines because they can form oil traps, where oil migrates up along the limbs of the fold and accumulates in the high point along the fold axis.
9.4.2 Syncline
3D Model 9.1: Synclinal fold
If you are using an offline version of this text, access this interactive 3D model via the QR code, or visit https://sketchfab.com/3d-models/synclinal-fold-macigno-formation-3f0259ea2c6b4807a32fe3c950d13324.
Synclines are troughlike, or U-shaped, folds that are concave-upward in shape. They have beds that dip down and in toward the central fold axis. In synclines, older rock is on the outside of the fold and the youngest rock is inside of the fold axis. A synform has the shape of a syncline but, like an antiform, does not have distinguishable age zones.
9.4.3 Monocline
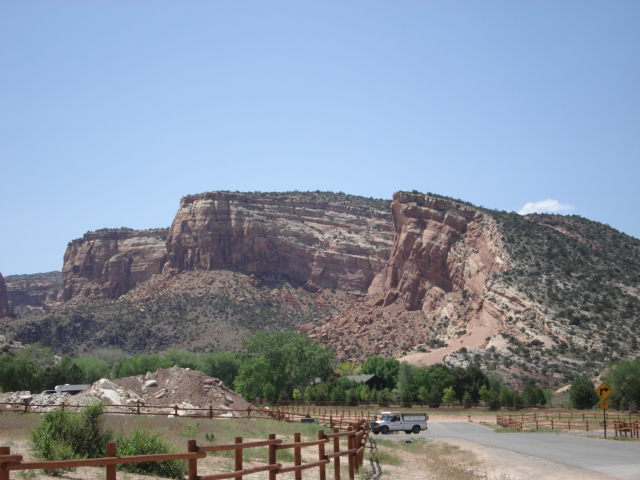
Monoclines are steplike folds in which flat rocks are upwarped or downwarped then continue flat. Monoclines are relatively common on the Colorado Plateau, where they form “reefs,” which are ridges that act as topographic barriers and should not be confused with ocean reefs (see Chapter 5). Capitol Reef is an example of a monocline in Utah. Monoclines can be caused by bending of shallower sedimentary strata as faults grow below them. These faults are commonly called “blind faults” because they end before reaching the surface and can be either normal or reverse faults.
9.4.4 Dome
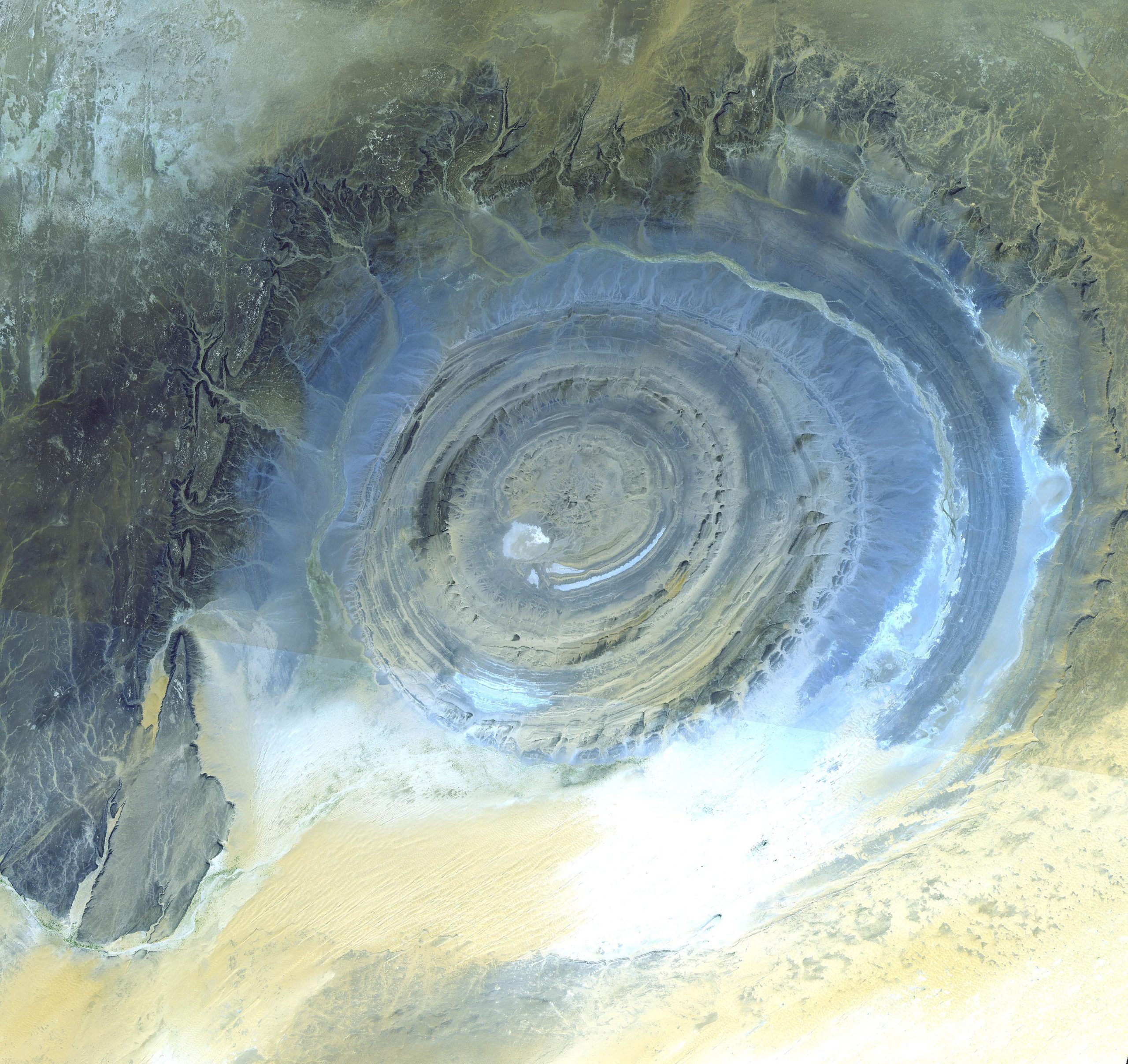
A dome is a symmetrical to semisymmetrical upwarping of rock beds. Domes have a shape like an inverted bowl, similar to an architectural dome on a building. Examples of domes in Utah include the San Rafael Swell, Harrisburg Junction Dome, and Henry Mountains. Domes are formed from compressional forces, underlying igneous intrusions (see Chapter 4), salt diapirs, or even impacts, like upheaval dome in Canyonlands National Park.
9.4.5 Basin
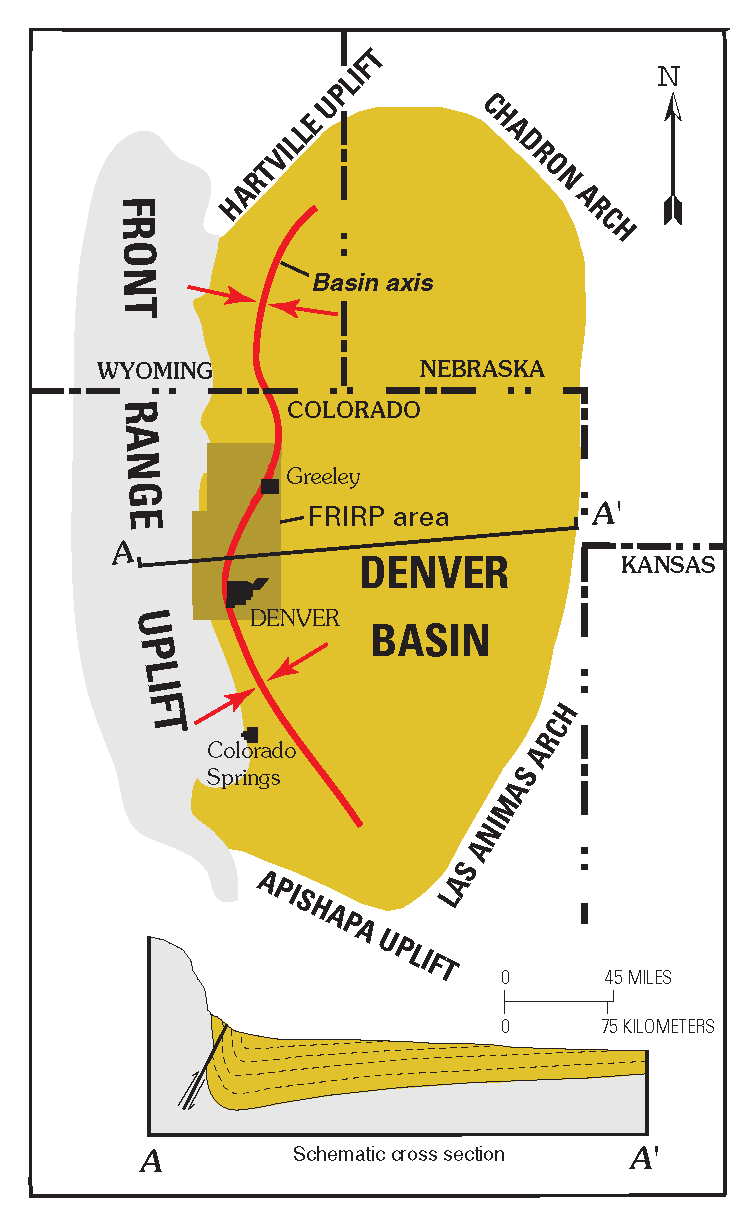
A basin is the inverse of a dome, a bowl-shaped depression in a rock bed. The Uinta Basin in Utah is an example of a basin. Some structural basins are also sedimentary basins that collect large quantities of sediment over time. Sedimentary basins can form as a result of folding but are much more commonly produced in mountain building, forming between mountain blocks or via faulting. Regardless of the cause, as the basin sinks or subsides, it can accumulate more sediment because the weight of the sediment causes more subsidence in a positive-feedback loop. There are active sedimentary basins all over the world. An example of a rapidly subsiding basin in Utah is the Oquirrh Basin, dated to the Pennsylvanian-Permian age, which has accumulated over 9,144 m (30,000 ft) of fossiliferous sandstones, shales, and limestones. These strata can be seen in the Wasatch Mountains along the east side of Utah Valley, especially on Mount Timpanogos and in Provo Canyon.
Take this quiz to check your comprehension of this section.
If you are using an offline version of this text, access the quiz for Section 9.4 via the QR code.
9.5 Faults
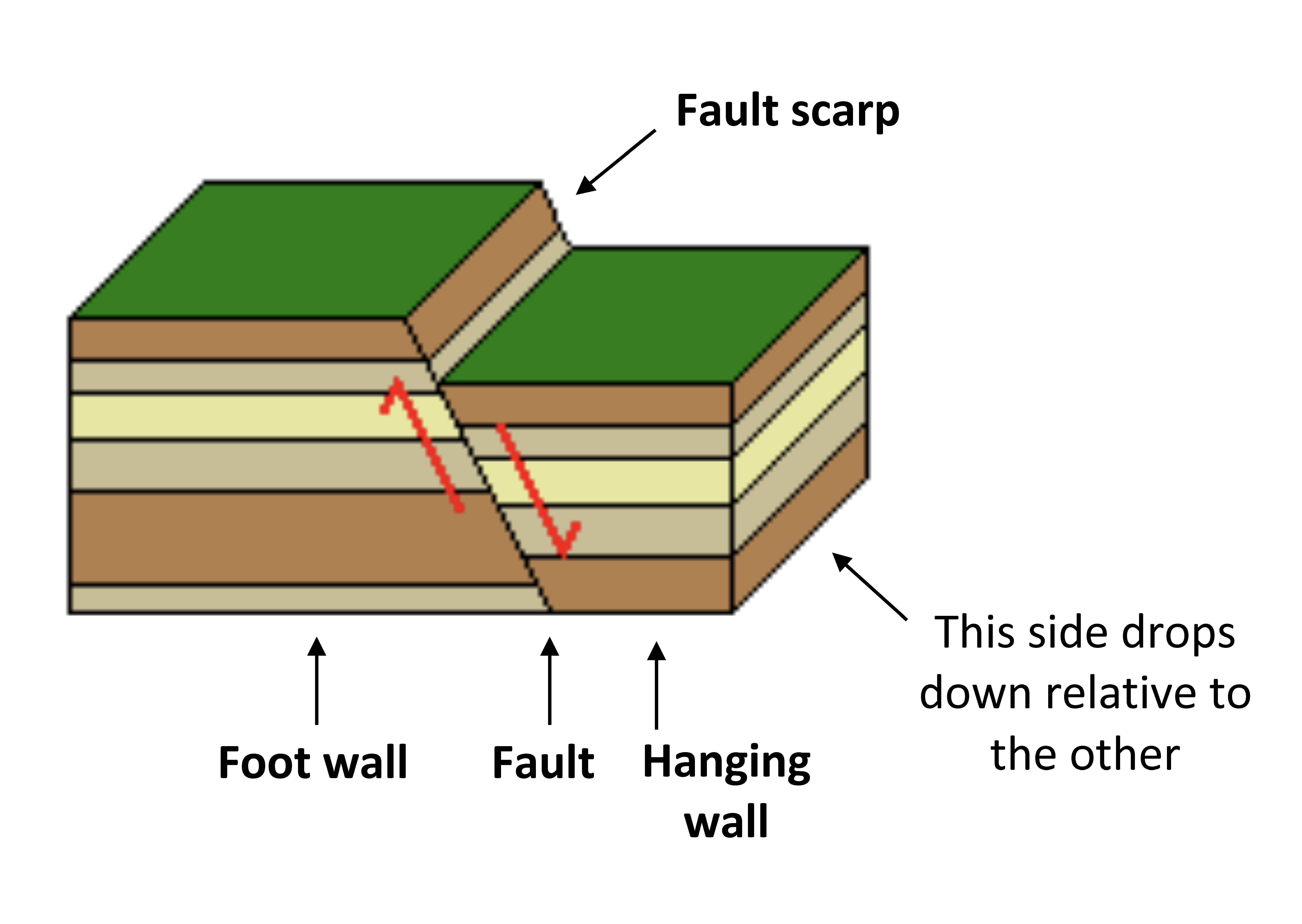
Faults are the places in the crust where brittle deformation occurs as two blocks of rocks move relative to one another. Normal and reverse faults display vertical, also known as dip-slip, motion. Dip-slip motion consists of relative up-and-down movement along a dipping fault between two blocks, the hanging wall and footwall. In a dip-slip system, the footwall is below the fault plane and the hanging wall is above the fault plane. A good way to remember this is to imagine a mine tunnel running along a fault; the hanging wall would be where a miner would hang a lantern, and the footwall would be at the miner’s feet.
Faulting as a term refers to the rupture of rocks. Such ruptures occur at plate boundaries but can also occur in plate interiors. Faults slip along the fault plane. The fault scarp is the offset of the surface produced by the fault breaking through the surface. Slickensides are polished, often grooved surfaces along the fault plane created by friction during the movement.
A joint or fracture is a plane of brittle deformation in rock created by movement that is not offset or sheared. Joints can result from many processes, such as cooling, depressurizing, or folding. Joint systems may be regional, affecting many square miles.
9.5.1 Normal Faults

Normal faults move by a vertical motion in which the hanging wall moves downward relative to the footwall along the dip of the fault. Normal faults are created by tensional forces in the crust. Normal faults and tensional forces commonly occur at divergent plate boundaries, where the crust is being stretched by tensional stresses (see Chapter 2). Examples of normal faults in Utah are the Wasatch fault, the Hurricane fault, and other faults bounding the valleys in the Basin and Range Province.
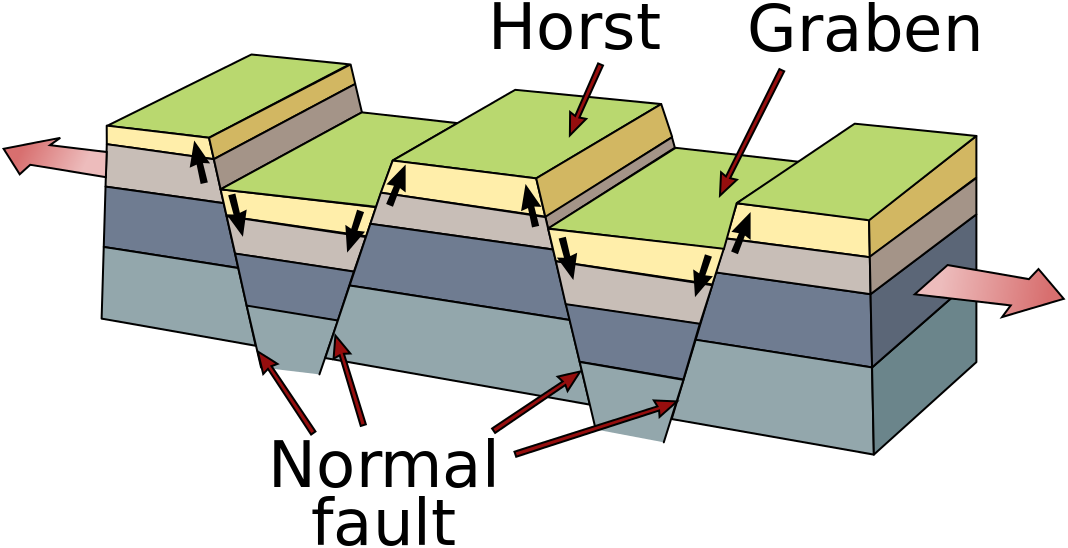
Grabens, horsts, and half-grabens are blocks of crust or rock bounded by normal faults (see Chapter 2). Grabens drop down relative to adjacent blocks and create valleys. Horsts rise up relative to adjacent down-dropped blocks and become areas of higher topography. In places where they occur together, horsts and grabens create a symmetrical pattern of valleys surrounded mountains and normal faults on both sides. Half-grabens are a one-sided version of a horst and graben, where blocks are tilted by a normal fault on one side, creating an asymmetrical valley-mountain arrangement. The mountain-valleys of the Basin and Range Province of Western Utah and Nevada consist of a series of full and half-grabens from the Salt Lake Valley to the Sierra Nevada Mountains.
Normal faults do not continue clear into the mantle. In the Basin and Range Province, the dip of a normal fault tends to decrease with depth, i.e., the fault angle becomes shallower and more horizontal as it goes deeper. Such decreasing dips happen when large amounts of extension occur along very low-angle normal faults, known as detachment faults. The normal faults of the Basin and Range, produced by tension in the crust, appear to become detachment faults at greater depths.
9.5.2 Reverse Faults
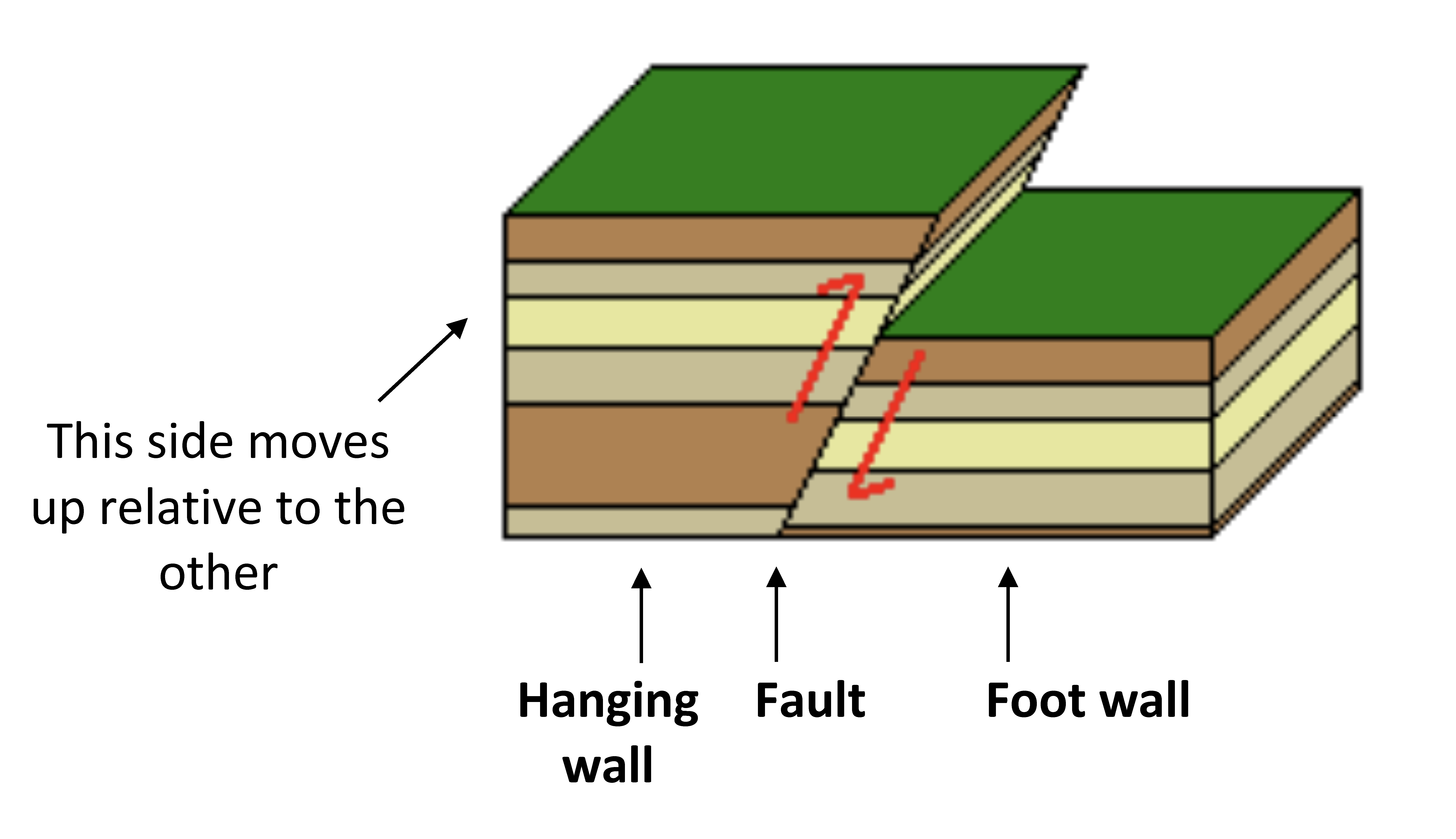
In reverse faults, compressional forces cause the hanging wall to move up relative to the footwall. A thrust fault is a reverse fault where the fault plane has a low dip angle of less than 45°. Thrust faults carry older rocks on top of younger rocks and can even cause repetition of rock units in the stratigraphic record.
Convergent plate boundaries with subduction zones create a special type of “reverse” fault called a megathrust fault, where denser oceanic crust drives down beneath less-dense overlying crust. Megathrust faults cause the largest magnitude earthquakes ever measured and commonly cause massive destruction and tsunamis.
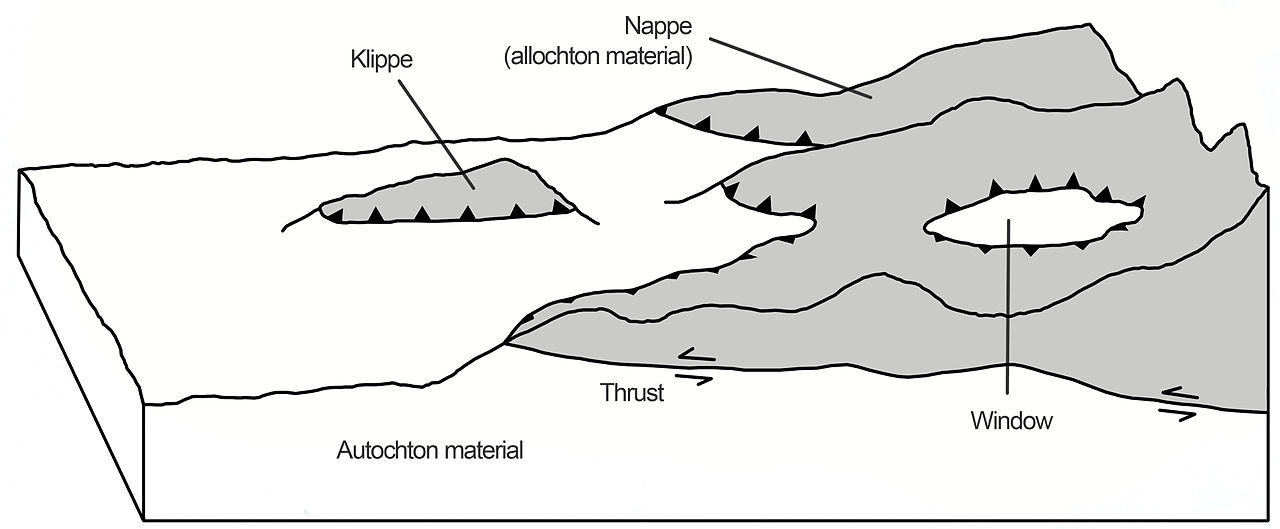
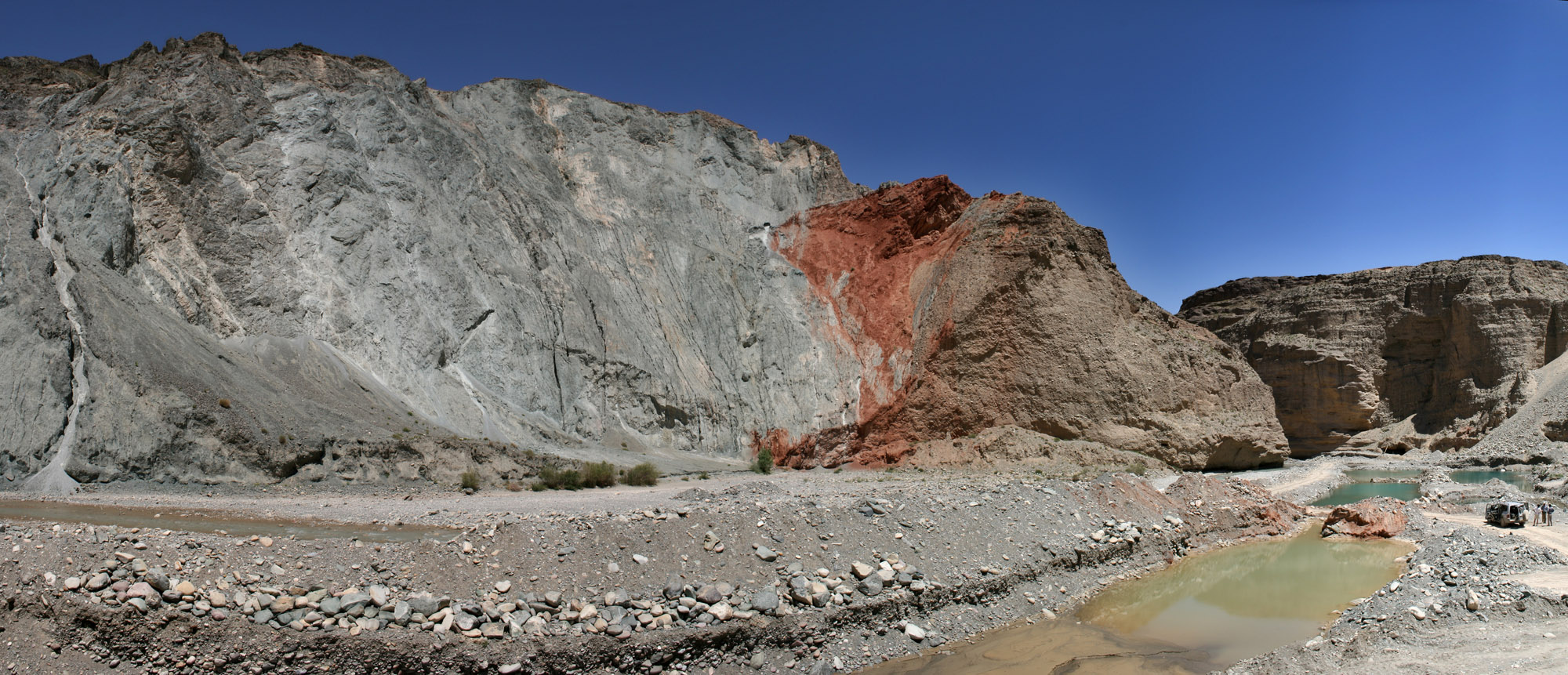
9.5.3 Strike–Slip Faults
Strike-slip faults have side-to-side motion. Strike-slip faults are most commonly associated with transform plate boundaries and are prevalent in transform fracture zones along mid-ocean ridges. In pure strike-slip motion, fault blocks on either side of the fault do not move up or down relative to each other; rather, they move laterally, side to side. The direction of strike-slip movement is determined by an observer standing on a block on one side of the fault. If the block on the opposing side of the fault moves left relative to the observer’s block, this is called sinistral motion. If the opposing block moves right, it is dextral motion.
Video 9.2: Video showing motion in a strike-slip fault
If you are using an offline version of this text, access this YouTube video via the QR code.
Bends along strike-slip faults create areas of compression or tension between the sliding blocks (see Chapter 2). Tensional stresses create transtensional features with normal faults and basins, such as the Salton Sea in California. Compressional stresses create transpressional features with reverse faults and cause small-scale mountain building, such as the San Gabriel Mountains in California. The faults that splay off transpression or transtension features are known as flower structures.
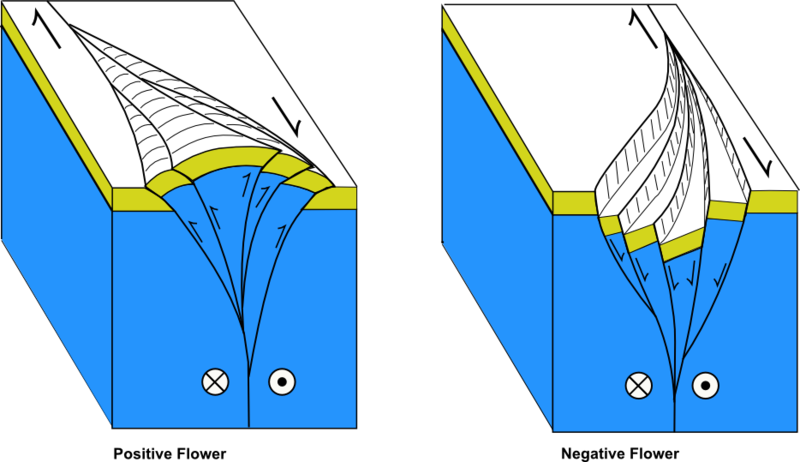
An example of a dextral, right-lateral strike-slip fault is the San Andreas fault, which denotes a transform boundary between the North American and Pacific plates. An example of a sinistral, left-lateral strike-slip fault is the Dead Sea fault in Jordan and Israel.
Video 9.3: Video showing how faults are classified
If you are using an offline version of this text, access this YouTube video via the QR code.
Take this quiz to check your comprehension of this section.
If you are using an offline version of this text, access the quiz for Section 9.5 via the QR code.
9.6 Earthquake Essentials
Earthquakes are felt at the surface of the Earth when energy is released by blocks of rock sliding past each other, evidence that faulting has occurred. Seismic energy thus released travels through the Earth in the form of seismic waves. Most earthquakes occur along active plate boundaries, but intraplate earthquakes (not along plate boundaries) occur and are still poorly understood. The USGS Earthquakes Hazards Program has a real-time map showing the most recent earthquakes.
9.6.1 How Earthquakes Happen
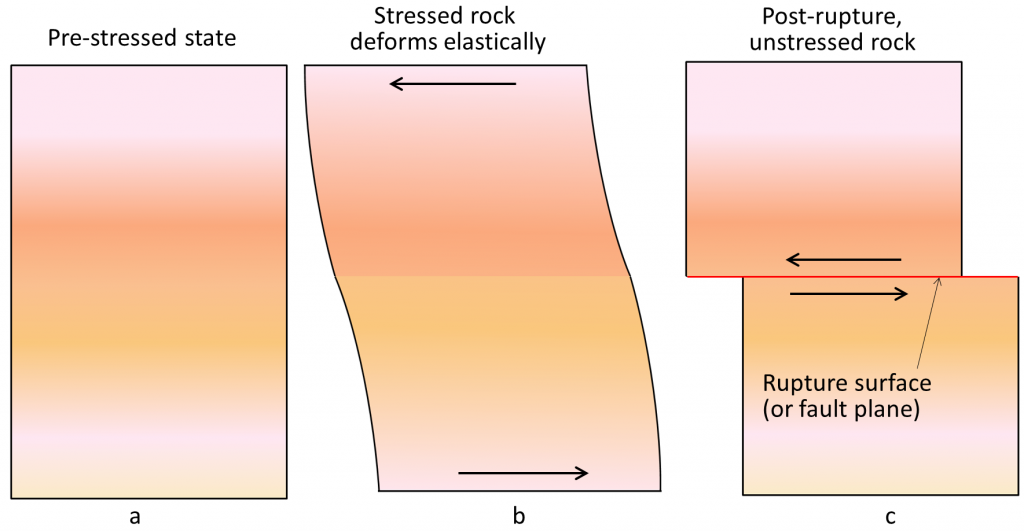
The release of seismic energy is explained by the elastic rebound theory. When rock is strained to the point that it undergoes brittle deformation, the place where the initial offsetting rupture takes place between the fault blocks is called the focus. This offset propagates along the fault, which is known as the fault plane.
The fault blocks of persistent faults like the Wasatch fault (Utah), showing recurring movements, are locked together by friction. Over hundreds to thousands of years, stress builds up along the fault until it overcomes frictional resistance, rupturing the rock and initiating fault movement. The deformed unbroken rocks snap back toward their original shape in a process called elastic rebound. Think of bending a stick until it breaks; stored energy is released, and the broken pieces return to near their original orientation.
Bending, the ductile deformation of the rocks near a fault, reflects a buildup of stress. In earthquake-prone areas like California, strain gauges are used to measure this bending and help seismologists, scientists who study earthquakes, understand more about predicting them. In locations where the fault is not locked, seismic stress causes continuous, gradual displacement between the fault blocks, which is called fault creep. Fault creep occurs along some parts of the San Andreas fault (California).
After an initial earthquake, continuous application of stress in the crust causes elastic energy to begin to build again during a period of inactivity along the fault. The accumulating elastic strain may be periodically released to produce small earthquakes called foreshocks on or near the main fault. Foreshocks can occur hours or days before a large earthquake or may not occur at all. The main release of energy during the major earthquake is known as the mainshock. Aftershocks may follow the mainshock to adjust new strain produced during the fault movement and generally decrease over time.
9.6.2 Focus and Epicenter
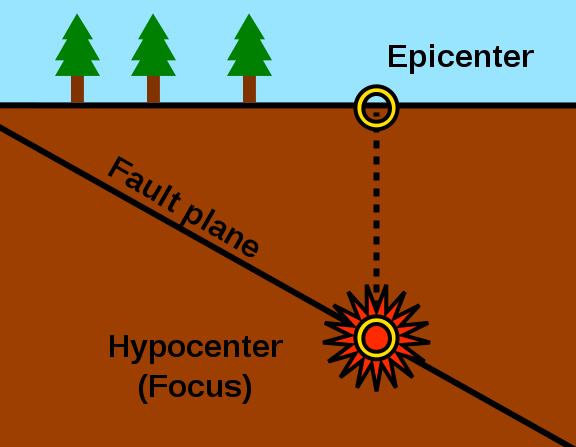
The earthquake focus, also called hypocenter, is the initial point of rupture, and displacement of the rock moves from the hypocenter along the fault surface. The earthquake focus is the point along the fault plane from which initial seismic waves spread outward and is always located at some depth below the ground surface. From the focus, rock displacement propagates up, down, and laterally along the fault plane. This displacement produces shock waves called seismic waves. The larger the displacement between the opposing fault blocks and the further the displacement propagates along the fault surface, the more seismic energy is released and the greater the amount and duration of shaking. The epicenter is the location on the Earth’s surface vertically above the focus. This is the location that most news reports give because it is the center of the area where people are affected.
9.6.3 Seismic Waves
To understand earthquakes and how earthquake energy moves through the Earth, consider the basic properties of waves. Waves describe how energy moves through a medium, such as rock or unconsolidated sediments in the case of earthquakes. Wave amplitude indicates the magnitude or height of earthquake motion. Wavelength is the distance between two successive peaks of a wave. Wave frequency is the number of repetitions of the motion over a period of time in the form of cycles per time unit. Period, which is the amount of time a wave takes to travel one wavelength, is the inverse of frequency. When multiple waves combine, they can interfere with each other (see Figure 9.19). When waves combine in sync, they produce constructive interference, where the influence of one wave adds to and magnifies the other. If waves are out of sync, they produce destructive interference, which diminishes the amplitudes of both waves. If two combined waves have the same amplitude and frequency but are one half-wavelength out of sync, the resulting destructive interference can eliminate each wave. These processes of wave amplitude, frequency, period, and constructive and destructive interference determine the magnitude and intensity of earthquakes.
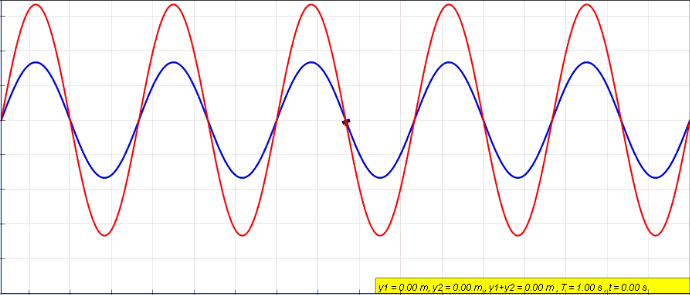
Seismic waves are the physical expression of energy released by the elastic rebound of rock within displaced fault blocks and are felt as an earthquake. Seismic waves occur as body waves and surface waves. Body waves pass underground through the Earth’s interior body and are the first seismic waves to propagate out from the focus. Body waves include primary (P) waves and secondary (S) waves. P waves are the fastest body waves and move through rock via compression, very much like sound waves move through air. Rock particles move forward and back during passage of the P waves, enabling them to travel through solids, liquids, plasma, and gases. S waves travel more slowly, following P waves, and propagate as shear waves that move rock particles from side to side. Because they are restricted to lateral movement, S waves can only travel through solids but not liquids, plasma, or gases.
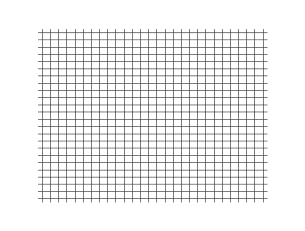
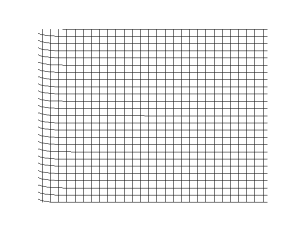
During an earthquake, body waves pass through the Earth and into the mantle as a subspherical wave front. Considering a point on a wave front, the path followed by a specific point on the spreading wave front is called a seismic ray, and a seismic ray reaches a specific seismograph located at one of thousands of seismic monitoring stations scattered over the Earth. Density increases with depth in the Earth, and since seismic velocity increases with density, a process called refraction causes earthquake rays to curve away from the vertical and bend back toward the surface, passing through different bodies of rock along the way.
Surface waves are produced when body waves from the focus strike the Earth’s surface. Surface waves travel along the Earth’s surface, radiating outward from the epicenter. Surface waves take the form of rolling waves called Rayleigh waves [watch wave propagation animation video] and side-to-side waves called Love waves [watch wave propagation animation video]. Surface waves are produced primarily as the more energetic S waves strike the surface from below, with some surface wave energy contributed by P waves. Surface waves travel more slowly than body waves, and because of their complex horizontal and vertical movement, surface waves are responsible for most of the damage caused by an earthquake. Love waves produce predominantly horizontal ground shaking and, ironically for their name, are the most destructive. Rayleigh waves produce an elliptical motion with longitudinal dilation and compression, like ocean waves. However, Rayleigh waves cause rock particles to move in a direction opposite to that of water particles in ocean waves.
The Earth has been described as ringing like a bell after an earthquake, with earthquake energy reverberating inside it. Like other waves, seismic waves refract (bend) and bounce (reflect) when passing through rocks of differing densities. S waves, which cannot move through liquid, are blocked by the Earth’s liquid outer core, creating an S wave shadow zone on the side of the planet opposite to the earthquake focus. P waves, on the other hand, pass through the core but are refracted into the core by the difference of density at the core-mantle boundary. This has the effect of creating a cone-shaped P wave shadow zone on parts of the other side of the Earth from the focus.
Video 9.4: Body and surface waves of 2011 Tohoku earthquake
If you are using an offline version of this text, access this video via the QR code.
9.6.4 Induced Seismicity
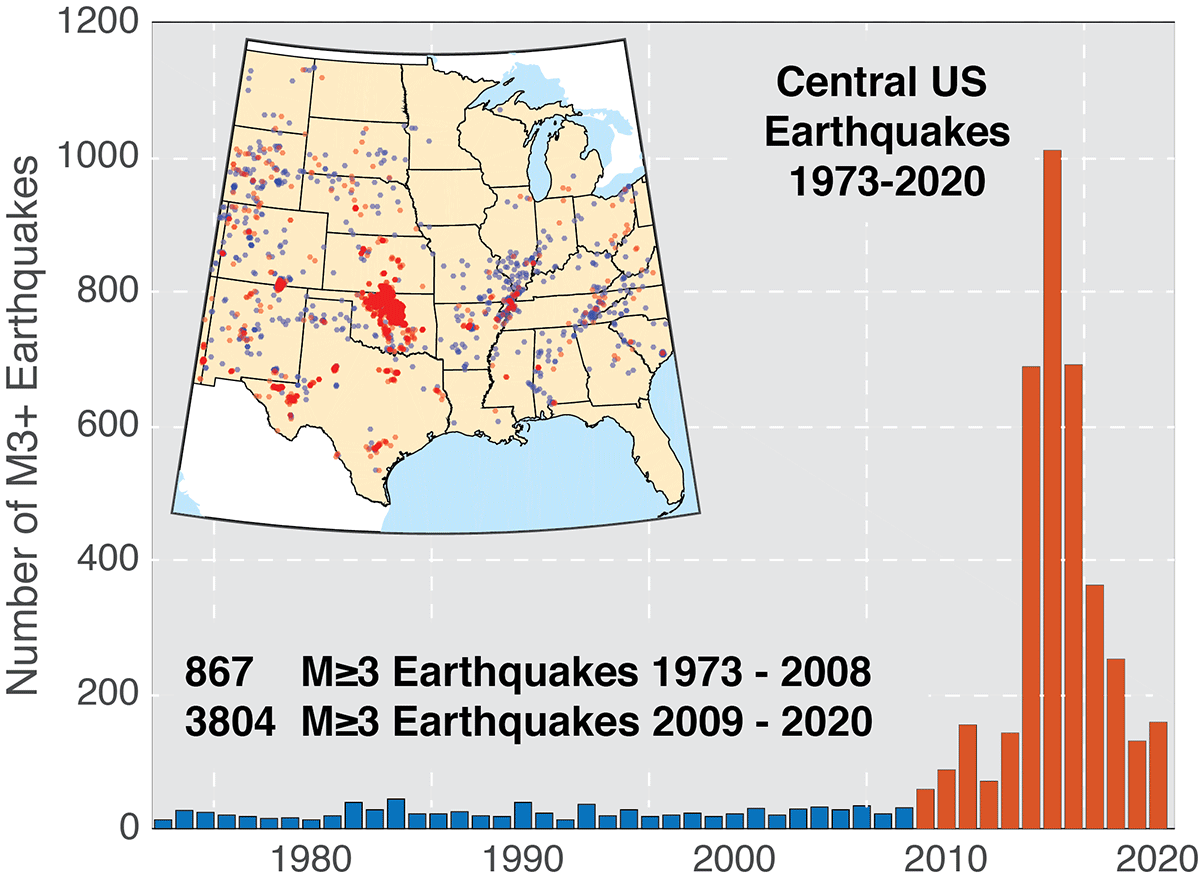
Earthquakes known as induced seismicity occur near natural gas extraction sites because of human activity. Injection of waste fluids in the ground, commonly a byproduct of an extraction process for natural gas known as fracking, can increase the outward pressure that liquid in the pores of a rock exerts, known as pore pressure. The increase in pore pressure decreases the frictional forces that keep rocks from sliding past each other, essentially lubricating fault planes. This effect is causing earthquakes to occur near injection sites in a human-induced activity known as induced seismicity. The significant increase in drilling activity in the Central United States has spurred the requirement for the disposal of significant amounts of waste drilling fluid, resulting in a measurable change in the cumulative number of earthquakes experienced in the region.
Take this quiz to check your comprehension of this section.
If you are using an offline version of this text, access the quiz for Section 9.6 via the QR code.
9.7 Measuring Earthquakes
9.7.1 Seismographs
Video 9.5: Animation of a horizontal seismograph
If you are using an offline version of this text, access this YouTube video via the QR code.
People feel approximately one million earthquakes a year, usually when they are close to the source and the earthquake registers a moment magnitude of at least 2.5. Major earthquakes of moment magnitude 7.0 and higher are extremely rare. The US Geological Survey (USGS) Earthquakes Hazards Program real-time map shows the location and magnitude of recent earthquakes around the world.
To accurately study seismic waves, geologists use seismographs that can measure even the slightest ground vibrations. Early twentieth-century seismograms use a weighted pen (pendulum) suspended by a long spring above a recording device fixed solidly to the ground. The recording device is a rotating drum mounted with a continuous strip of paper. During an earthquake, the suspended pen stays motionless and records ground movement on the paper strip. The resulting graph is known as a seismogram. Digital versions use magnets, wire coils, electrical sensors, and digital signals instead of mechanical pens, springs, drums, and paper. A seismograph array is multiple seismographs configured to measure vibrations in three directions: north-south (x-axis), east-west (y-axis), and up-down (z-axis).
Video 9.6: Animation of a vertical seismograph
If you are using an offline version of this text, access this YouTube video via the QR code.
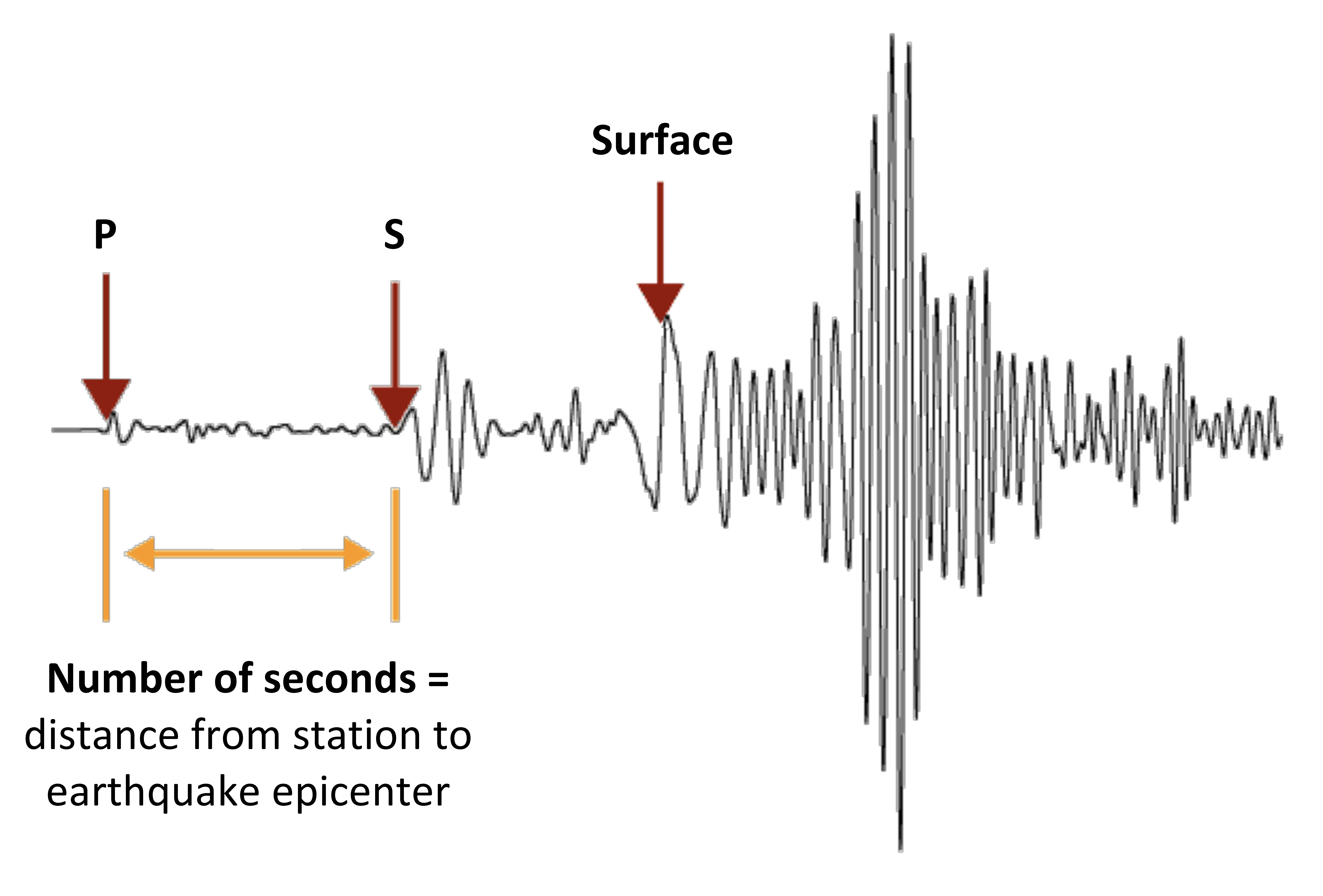
To pinpoint the location of an earthquake epicenter, seismologists use the differences in arrival times of the P, S, and surface waves. After an earthquake, P waves will appear first on a seismogram, followed by S waves, and finally surface waves, which have the largest amplitude. It is important to note that surface waves lose energy quickly, so they are not measurable at great distances from the epicenter. These time differences determine the distance but not the direction of the epicenter. By using wave arrival times recorded on seismographs at multiple stations, seismologists can apply triangulation to pinpoint the location of the epicenter of an earthquake. At least three seismograph stations are needed for triangulation. The distance from each station to the epicenter is plotted as the radius of a circle. The epicenter is demarked where the circles intersect. This method also works in 3-D, using multi-axis seismographs and sphere radii to calculate the underground depth of the focus.
Video 9.7: Method of triangulation used to locate the epicenter of an earthquake
If you are using an offline version of this text, access this YouTube video via the QR code.
9.7.2 Seismograph Network
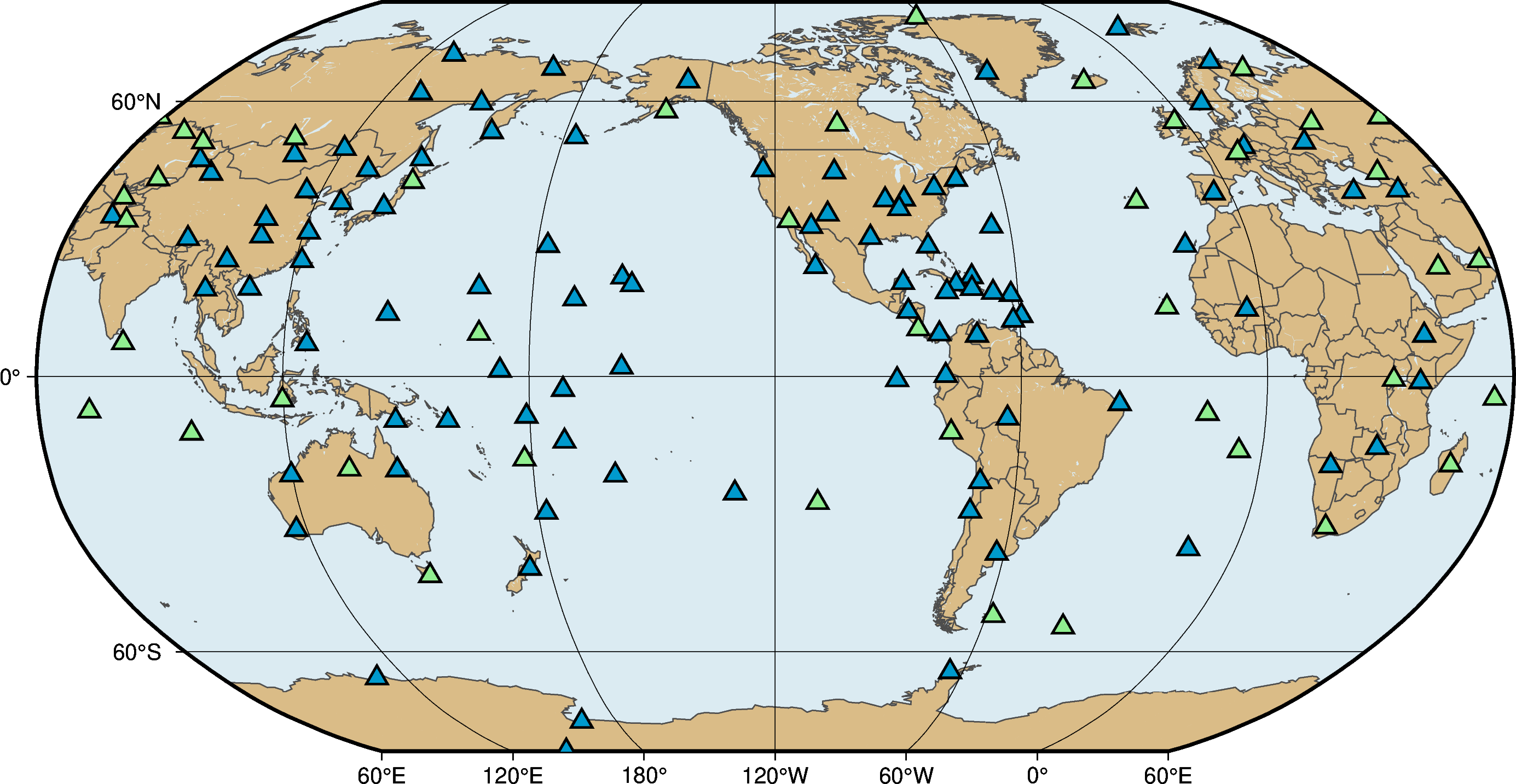
The International Registry of Seismograph Stations lists more than 20,000 seismographs on the planet. By comparing data from multiple seismographs, scientists can map the properties of the inside of the Earth, detect detonations of large explosive devices, and predict tsunamis. The Global Seismic Network, a worldwide set of linked seismographs that electronically distribute real-time data, includes more than 150 stations that meet specific design and precision standards. The USArray is a network of hundreds of permanent and transportable seismographs in the United States that are used to map the subsurface activity of earthquakes (see Video 9.8).
Along with monitoring for earthquakes and related hazards, the Global Seismograph Network helps detect nuclear weapons testing, which is monitored by the Comprehensive Nuclear Test Ban Treaty Organization. Most recently, seismographs have been used to determine nuclear weapons testing by North Korea.
Video 9.8: Nepal earthquake (M7.9) ground motion visualization
If you are using an offline version of this text, access this YouTube video via the QR code.
9.7.3 Seismic Tomography
Very much like a CT (computed tomography) scan uses X-rays at different angles to image the inside of a body, seismic tomography uses seismic rays from thousands of earthquakes that occur each year, passing at all angles through masses of rock, to generate images of internal Earth structures.
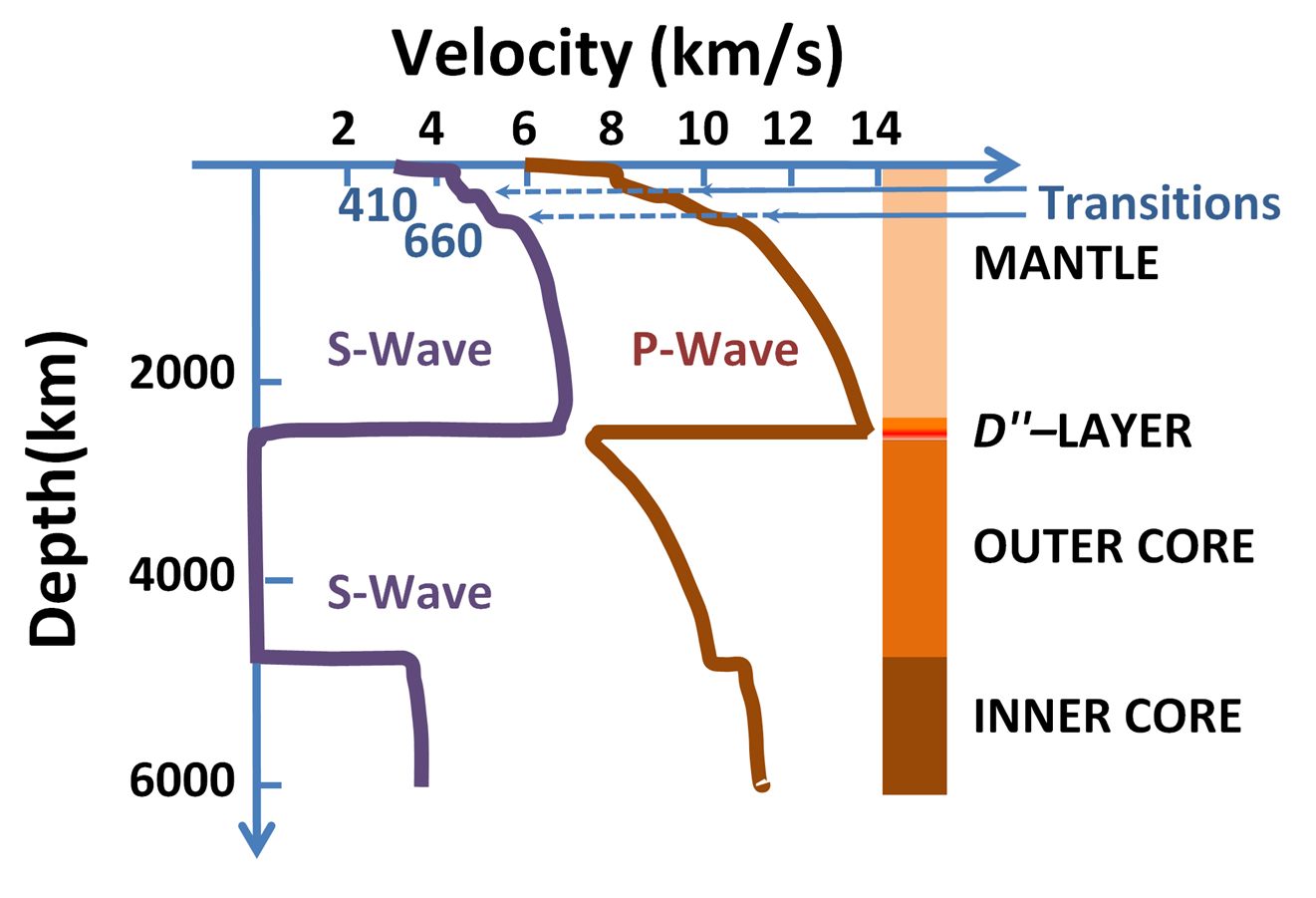
Using the assumption that the Earth consists of homogenous layers, geologists developed a model of expected properties of Earth materials at every depth called the PREM (preliminary reference earth model). These properties include seismic wave transmission velocity, which is dependent on rock density and elasticity. In the mantle, temperature differences affect rock density. Cooler rocks have a higher density and therefore transmit seismic waves more quickly. Warmer rocks have a lower density and transmit earthquake waves more slowly. When the arrival times of seismic rays at individual seismic stations are compared to arrival times predicted by PREM, differences are called seismic anomalies and can be measured for bodies of rock within the Earth from seismic rays passing through them at seismic network stations. Because seismic rays travel at all angles from many earthquakes and arrive at many stations, variations in the properties of the rock bodies allow 3-D images to be constructed of the rock bodies through which the rays passed. Seismologists are thus able to construct 3-D images of the interior of the Earth.
For example, seismologists have mapped the Farallon plate, a tectonic plate that subducted beneath North America during the last several million years, and the Yellowstone magma chamber, which is a product of the Yellowstone hotspot under the North American continent. Peculiarities of the Farallon plate subduction are thought to be responsible for many features of western North America, including the Rocky Mountains (see Chapter 8).
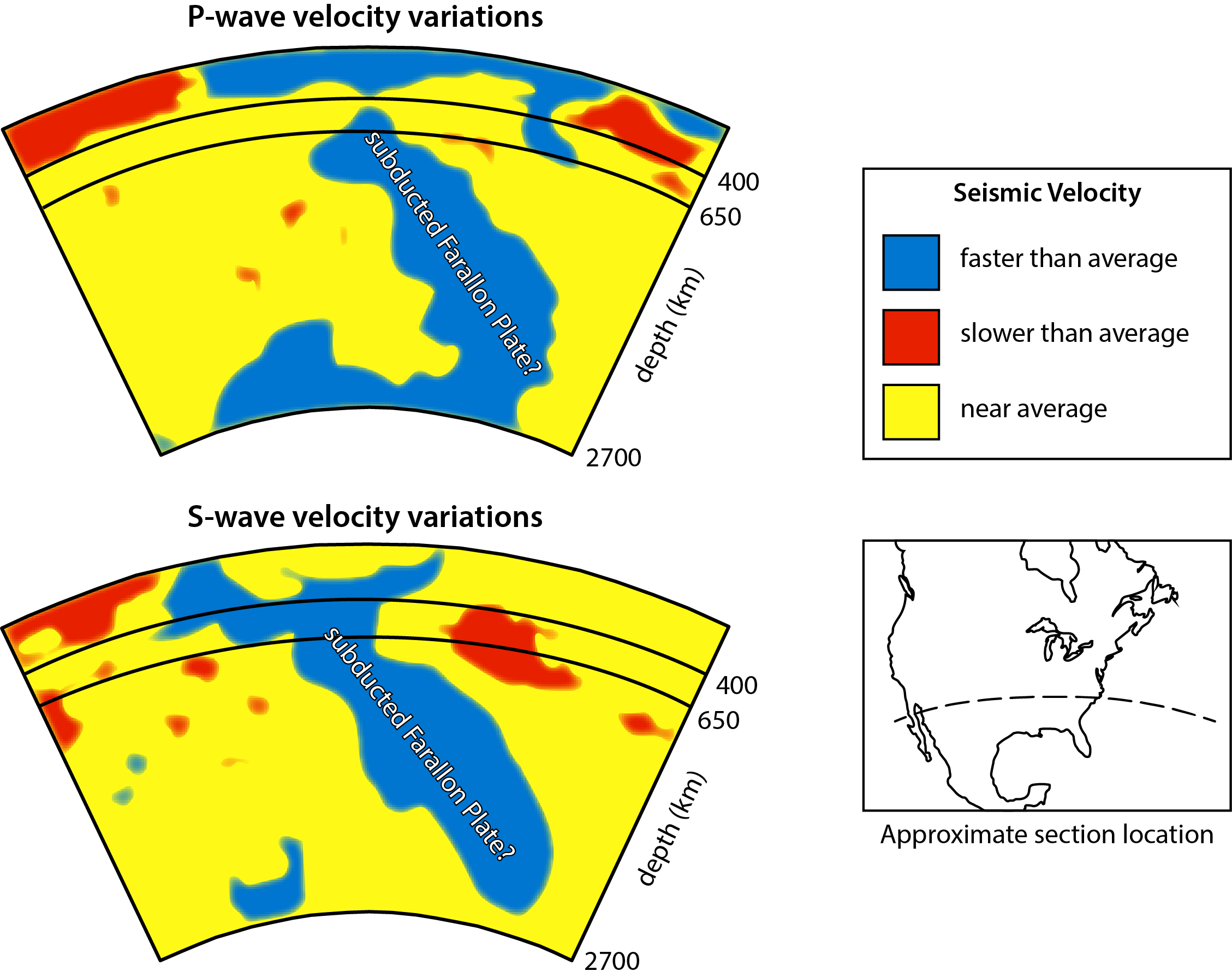
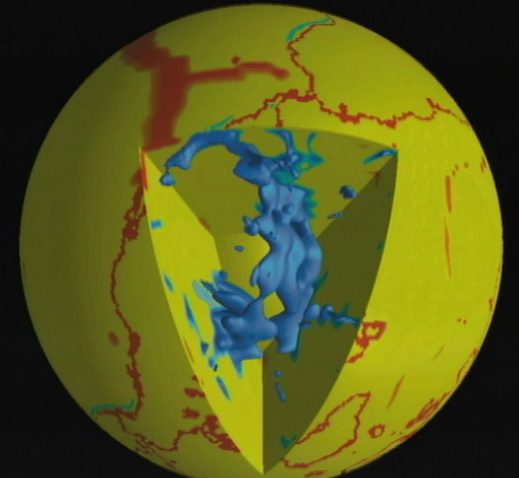
9.7.4 Earthquake Magnitude and Intensity
Richter Scale
Magnitude is the measure of the energy released by an earthquake. The Richter scale, the first and most well-known magnitude scale, was developed by Charles F. Richter (1900–1985) at the California Institute of Technology. This was the magnitude scale used historically by early seismologists. Richter magnitude (ML) is determined from the maximum amplitude of the pen tracing on the seismogram recording. Adjustments for epicenter distance from the seismograph are made using the arrival-time differences of S and P waves.
The Richter scale is logarithmic, based on powers of 10. This means an increase of one Richter unit represents a tenfold increase in seismic-wave amplitude; in other words, a magnitude 6 earthquake shakes the ground ten times more than a magnitude 5. However, the actual energy released for each magnitude unit is 32 times greater, which means a magnitude 6 earthquake releases 32 times more energy than a magnitude 5.
The Richter scale was developed for earthquakes in Southern California, using local seismographs. It has limited applications for larger distances and very large earthquakes. Therefore, most agencies no longer use the Richter scale. Moment magnitude (MW), which is measured using seismic arrays and generates values comparable to the Richter scale, is more accurate for measuring earthquakes across the Earth, including large earthquakes, although they require more time to calculate. News media often report Richter magnitudes right after an earthquake occurs, even though scientific calculations now use moment magnitudes.
Moment Magnitude Scale
The moment magnitude scale depicts the absolute size of earthquakes, comparing information from multiple locations and using a measurement of actual energy released calculated from the cross-sectional area of rupture, amount of slippage, and the rigidity of the rocks. Because each earthquake occurs in a unique geologic setting and the rupture area is often hard to measure, estimates of moment magnitude can take days or even months to calculate.
Like the Richter scale, the moment magnitude scale is logarithmic. Magnitude values of the two scales are approximately equal, except for very large earthquakes. Both scales are used for reporting earthquake magnitude. The Richter scale provides a quick magnitude estimate immediately following the quake. Moment magnitude calculations take much longer but are more accurate and thus, more useful for scientific analysis.
Video 9.9: Moment magnitude explained
If you are using an offline version of this text, access this YouTube video via the QR code.
Modified Mercalli Intensity Scale
The modified Mercalli intensity (MMI) scale is a qualitative rating of ground-shaking intensity based on observable structural damage and people’s perceptions. This scale uses a I (Roman numeral one) rating for the lowest intensity and X (ten) for the highest (see Table 9.3) and can vary depending on epicenter location and population density, such as urban versus rural settings. Historically, scientists used the MMI scale to categorize earthquakes before they developed quantitative measurements of magnitude. Intensity maps show locations of the most severe damage based on residential questionnaires, local news articles, and onsite assessment reports.
Intensity | Shaking | Description/damage |
---|---|---|
I | Not felt | Not felt except by a very few under especially favorable conditions. |
II | Weak | Felt only by a few persons at rest, especially on upper floors of buildings. |
III | Weak | Felt quite noticeably by persons indoors, especially on upper floors of buildings. Many people do not recognize it as an earthquake. Standing motor cars may rock slightly. Vibrations similar to the passing of a truck. Duration estimated. |
IV | Light | Felt indoors by many, outdoors by few during the day. At night, some awakened. Dishes, windows, doors disturbed; walls make cracking sound. Sensation like heavy truck striking building. Standing motor cars rocked noticeably. |
V | Moderate | Felt by nearly everyone; many awakened. Some dishes, windows broken. Unstable objects overturned. Pendulum clocks may stop. |
VI | Strong | Felt by all, many frightened. Some heavy furniture moved; a few instances of fallen plaster. Damage slight. |
VII | Very strong | Damage negligible in buildings of good design and construction; slight to moderate in well-built ordinary structures; considerable damage in poorly built or badly designed structures; some chimneys broken. |
VIII | Severe | Damage slight in specially designed structures; considerable damage in ordinary substantial buildings with partial collapse. Damage great in poorly built structures. Fall of chimneys, factory stacks, columns, monuments, walls. Heavy furniture overturned. |
IX | Violent | Damage considerable in specially designed structures; well-designed frame structures thrown out of plumb. Damage great in substantial buildings, with partial collapse. Buildings shifted off foundations. |
X | Extreme | Some well-built wooden structures destroyed; most masonry and frame structures destroyed with foundations. Rails bent. |
Table 9.3: Abridged Mercalli scale. (Source: USGS General Interest Publication. 1989, 288-913.)
Shake Maps
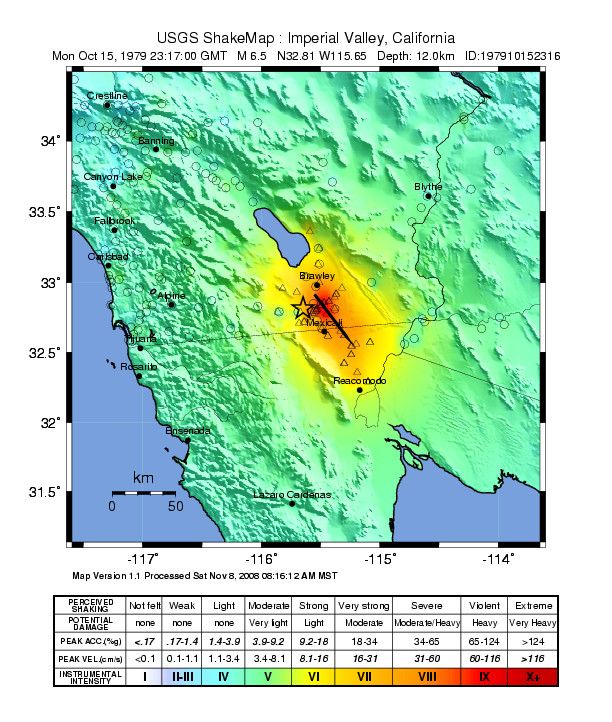
Shake maps, written ShakeMaps by the USGS, use high-quality, computer-interpolated data from seismograph networks to show areas of intense shaking. Shake maps are useful in the crucial minutes after an earthquake, as they show emergency personnel where the greatest damage likely occurred and help them locate possibly damaged gas lines and other utility facilities.
Take this quiz to check your comprehension of this section.
If you are using an offline version of this text, access the quiz for Section 9.7 via the QR code.
9.8 Earthquake Risk
9.8.1 Factors That Determine Shaking
Earthquake magnitude is an absolute value that measures pure energy release. However, intensity (i.e., how much the ground shakes) is determined by several factors.
Earthquake magnitude: In general, the larger the magnitude, the stronger the shaking and the longer the shaking will last.
This table is taken from the USGS and shows scales of magnitude and Mercalli intensity, as well as descriptions of shaking and resulting damage.
Magnitude | Modified Mercalli intensity | Shaking/damage description |
---|---|---|
1.0-3.0 | I | Only felt by a very few. |
3.0-3.9 | II-III | Noticeable indoors, especially on upper floors. |
4.0-4.9 | IV-V | Most to all feel it. Dishes, doors, cars shake and possibly break. |
5.0-5.9 | VI-VII | Everyone feels it. Some items knocked over or broken. Building damage possible. |
6.0-6.9 | VII-IX | Frightening amounts of shaking. Significant damage especially with poorly constructed buildings. |
≥ 7.0 | ≥ VIII | Significant destruction of buildings. Potential for objects to be thrown in air from shaking. |
Table 9.4: Mercalli intensity as it relates to magnitude.
Location and direction: Shaking is more severe closer to the epicenter. The severity of shaking is influenced by the location of the observer relative to epicenter, direction of rupture propagation, and path of greatest rupture.
Local geologic conditions: Seismic waves are affected by the nature of the ground materials through which they pass. Different materials respond differently to an earthquake. Think of shaking a block of Jell-O versus a meatloaf—one will jiggle much more when hit by waves of the same amplitude. The ground’s response to shaking depends on the degree of substrate consolidation. Solid sedimentary, igneous, or metamorphic bedrock shakes less than unconsolidated sediments.
Video 9.10: How different substrates behave in response to different seismic waves and their potential for destruction
If you are using an offline version of this text, access this YouTube video via the QR code.
Seismic waves move fastest through consolidated bedrock, slower through unconsolidated sediments, and slowest through unconsolidated sediments with a high water content. Seismic energy is transmitted by wave velocity and amplitude. When seismic waves slow down, energy is transferred to the amplitude, increasing the motion of surface waves, which in turn amplifies ground shaking.
Focus depth: Deeper earthquakes cause less surface shaking because much of their energy, transmitted as body waves, is lost before reaching the surface. Recall that surface waves are generated by P and S waves impacting the Earth’s surface.
9.8.2 Factors That Determine Destruction
Just as certain conditions will impact intensity of ground shaking, several factors affect how much destruction is caused.

Building materials: The flexibility of a building material determines its resistance to earthquake damage. Unreinforced masonry (URM) is the material most devastated by ground shaking. Wood framing fastened with nails bends and flexes during seismic wave passage and is more likely to survive intact. Steel also has the ability to deform elastically before brittle failure. The Fix the Bricks campaign in Salt Lake City, Utah, has good information on URMs and earthquake safety.
Intensity and duration: Greater shaking and duration of shaking causes more destruction than lower and shorter shaking.
Resonance: Resonance occurs when seismic wave frequency matches a building’s natural shaking frequency and increases the shaking. This happened in the 1985 Mexico City earthquake, where buildings of heights between six and 15 stories were especially vulnerable to earthquake damage. Skyscrapers designed with earthquake resilience have dampers and base isolation features to reduce resonance.
Resonance is influenced by the properties of the building materials. Changes in the structural integrity of a building can alter resonance. Conversely, changes in measured resonance can indicate a potentially altered structural integrity.
These two videos discuss why buildings fall during earthquakes and a modern procedure to reduce potential earthquake destruction for larger buildings.
Video 9.11: Why do buildings fall in earthquakes?
If you are using an offline version of this text, access this YouTube video via the QR code.
Video 9.12: Base isolators
If you are using an offline version of this text, access this YouTube video via the QR code.
9.8.3 Earthquake Recurrence
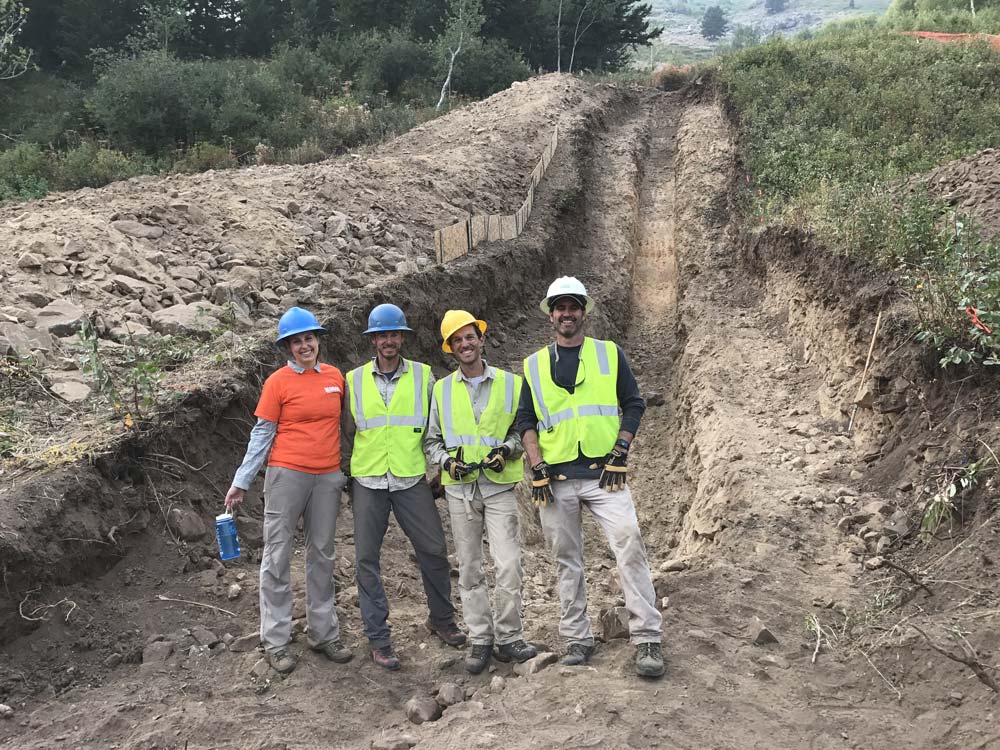
A long hiatus in activity along a fault segment with a history of recurring earthquakes is known as a seismic gap. The lack of activity may indicate the fault segment is locked, which may produce a buildup of strain and higher probability of an earthquake recurring. Geologists dig earthquake trenches across faults to estimate the frequency of past earthquake occurrences. Trenches are effective for faults with relatively long recurrence intervals of roughly 100s to 10,000s of years between significant earthquakes. Trenches are less useful in areas with more frequent earthquakes because they usually have more recorded data.
9.8.4 Earthquake Distribution
Like volcanoes, earthquakes tend to aggregate around active boundaries of tectonic plates. The exception is intraplate earthquakes, which are comparatively rare.
Below are five areas where different sizes of earthquakes occur.
Subduction zones: Subduction zones, found at convergent plate boundaries, are where megathrust earthquakes, the largest and deepest earthquakes, occur. Examples of subduction-zone earthquake areas include the Sumatran Islands, Aleutian Islands, west coast of South America, and Cascadia subduction zone off the coast of Washington and Oregon. See Chapter 2 for more information about subduction zones.
Collision zones: Collisions between converging continental plates create broad earthquake zones that may generate deep, large earthquakes from the remnants of past subduction events or other deep-crustal processes. The Himalayan Mountains (northern border of the Indian subcontinent) and Alps (southern Europe and Asia) are active regions of collision-zone earthquakes. See Chapter 2 for more information about collision zones.
Transform boundaries: Strike-slip faults created at transform boundaries produce moderate-to-large earthquakes that usually have a maximum moment magnitude of about 8. The San Andreas fault (California) is an example of a transform-boundary earthquake zone. Other examples of transform faults include Haiti’s Enriquillo-Plantain Garden fault system, which caused the 2010 earthquake near Port-au-Prince (see below), and Septentrional fault, which destroyed Cap-Haïtien in 1842 and shook Cuba in 2020, as well as the Alpine fault (New Zealand) and Anatolian faults (Turkey). See Chapter 2 for more information about transform boundaries.
Divergent boundaries: Continental rifts and mid-ocean ridges found at divergent boundaries generally produce moderate earthquakes. Examples of active earthquake zones include the East African Rift System (southwestern Asia through eastern Africa), Iceland, and Basin and Range Province (Nevada, Utah, California, Arizona, and northwestern Mexico). See Chapter 2 for more information about divergent boundaries.
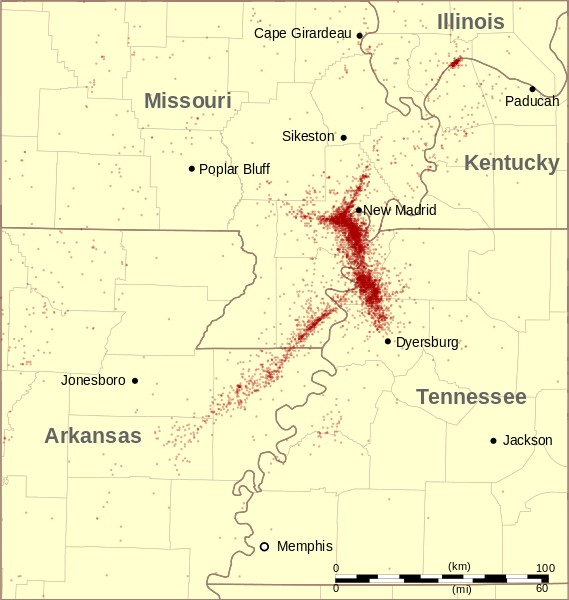
Intraplate earthquakes: Intraplate earthquakes are not found near tectonic plate boundaries but generally occur in areas of weakened crust or concentrated tectonic stress. The New Madrid seismic zone, which covers Missouri, Illinois, Tennessee, Arkansas, and Indiana, is thought to represent the failed Reelfoot rift. The failed rift zone weakened the crust, making it more responsive to tectonic plate movement and interaction. Geologists theorize the infrequently occurring earthquakes are produced by low strain rates
9.8.5 Secondary Hazards Caused by Earthquakes
Most earthquake damage is caused by ground shaking and fault block displacement. In addition, there are secondary hazards that endanger structures and people, in some cases after the shaking stops.
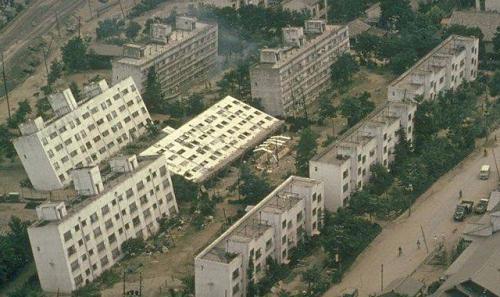
Buildings toppled from liquefaction during a 7.5 magnitude earthquake in Japan.
Liquefaction: Liquefaction occurs when water-saturated, unconsolidated sediments, usually silt or sand, become fluidlike from shaking. The shaking breaks the cohesion between grains of sediment, creating a slurry of particles suspended in water. Buildings settle or tilt in the liquified sediment, which looks very much like quicksand from the movies. Liquefaction also creates sand volcanoes, cone-shaped features created when liquefied sand is squirted through an overlying and usually finer-grained layer.
Video 9.13: How liquefaction takes place
If you are using an offline version of this text, access this YouTube video via the QR code.
This video shows liquefaction occurring during the 2011 earthquake in Japan.
Video 9.14: Liquefaction during the 2011 earthquake in Japan
If you are using an offline version of this text, access this YouTube video via the QR code.
Tsunamis: Among the most devastating natural disasters are tsunamis, earthquake-induced ocean waves. When the seafloor is offset by fault movement or an underwater landslide, the ground displacement lifts a volume of ocean water and generates the tsunami wave. Ocean wave behavior, which includes tsunamis, is covered in Chapter 12. Tsunami waves are fast-moving with low amplitude in deep ocean water, but their amplitude grows significantly in the shallower waters approaching shore. When a tsunami is about to strike land, the drawback of the trough preceding the wave crest causes the water to recede dramatically from shore. Tragically, curious people wander out and follow the disappearing water, only to be overcome by an oncoming wall of water that can be upwards of a 30 m (100 ft) high. Early warning systems help mitigate the loss of life caused by tsunamis.
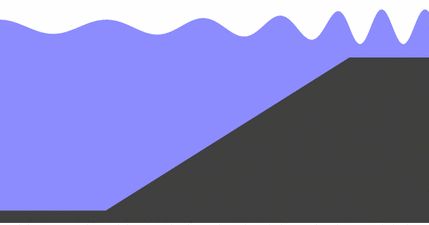
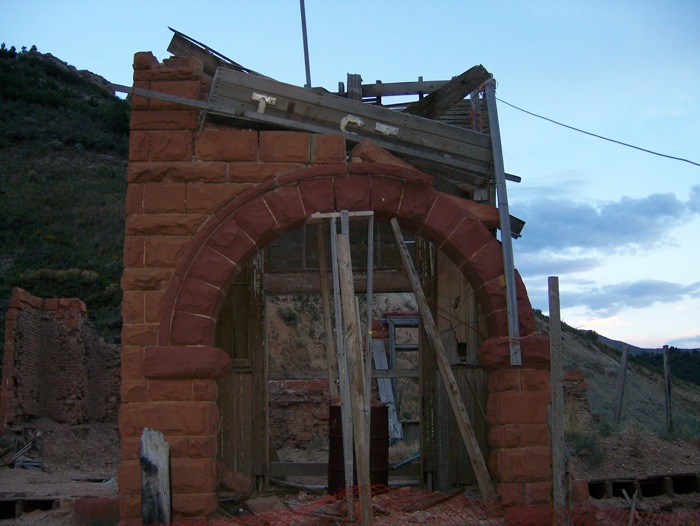
Landslides: Shaking can trigger landslides (see Chapter 10). In 1992, a moment magnitude 5.9 earthquake in St. George, Utah, caused a landslide that destroyed several structures in the Balanced Rock Hills subdivision in Springville, Utah.
Seiches: Seiches are waves generated in lakes by earthquakes. The shaking may cause water to slosh back and forth, or sometimes change the lake depth. Seiches in Hebgen Lake during a 1959 earthquake caused major destruction to nearby structures and roads.
This video shows a seich generated in a swimming pool by an earthquake in Nepal in 2015.
Video 9.15: A seich generated in a swimming pool by an earthquake in Nepal in 2015
If you are using an offline version of this text, access this YouTube video via the QR code.
Land elevation changes: Elastic rebound and displacement along the fault plane can cause significant land elevation changes, such as subsidence or upheaval. The 1964 Alaska earthquake produced significant land elevation changes, with the differences in height between the hanging wall and footwall ranging from one to several meters (3–30 ft). The Wasatch Mountains in Utah represent an accumulation of fault scarps created a few meters at a time, over a few million years.
Take this quiz to check your comprehension of this section.
If you are using an offline version of this text, access the quiz for Section 9.8 via the QR code.
9.9 Case Studies
Video 9.16 explains the seismic activity and hazards of the Intermountain seismic belt and the Wasatch fault, a large intraplate area of seismic activity.
Video 9.16: Activities of the Intermountain seismic belt and the Wasatch fault.
If you are using an offline version of this text, access this YouTube video via the QR code.
9.9.1 North American Earthquakes
Basin and Range earthquakes: Earthquakes in the Basin and Range Province, from the Wasatch fault (Utah) to the Sierra Nevada (California), occur primarily in normal faults created by tensional forces. The Wasatch fault, which defines the eastern extent of the Basin and Range Province, has been studied as an earthquake hazard for more than 100 years.
New Madrid earthquakes (1811–1812): Historical accounts of earthquakes in the New Madrid seismic zone date as far back as 1699, and earthquakes continue to be reported in modern times. A sequence of large (Mw >7) occurred from December 1811 to February 1812 in the New Madrid area of Missouri. The earthquakes damaged houses in St. Louis, affected the stream course of the Mississippi River, and leveled the town of New Madrid. These earthquakes were the result of intraplate seismic activity.
Charleston (1886): The 1886 earthquake in Charleston, South Carolina, was a moment magnitude 7.0, with a Mercalli intensity of X. It caused significant ground motion and killed at least 60 people. This intraplate earthquake was likely associated with ancient faults created during the breakup of Pangea. The earthquake caused significant liquefaction. Scientists estimate the recurrence of destructive earthquakes in this area with an interval of approximately 1,500 to 1,800 years.
Great San Francisco earthquake and eire (1906): On April 18, 1906, a large earthquake with an estimated moment magnitude of 7.8 and MMI of X occurred along the San Andreas fault near San Francisco, California. There were multiple aftershocks followed by devastating fires, resulting in about 80% of the city being destroyed. Geologists G.K. Gilbert and Richard L. Humphrey, working independently, arrived the day following the earthquake and took measurements and photographs.

Alaska (1964): The 1964 Alaska earthquake, moment magnitude 9.2, was one of the most powerful earthquakes ever recorded. The earthquake originated in a megathrust fault along the Aleutian subduction zone. The earthquake caused large areas of land subsidence and uplift, as well as significant mass wasting.
The following video from the USGS describes the 1964 Alaska earthquake.
Video 9.17: The 1964 Alaska earthquake
If you are using an offline version of this text, access this YouTube video via the QR code.
Loma Prieta (1989): The Loma Prieta, California, earthquake was created by movement along the San Andreas fault. The moment magnitude 6.9 earthquake was followed by a magnitude 5.2 aftershock. It caused 63 deaths, buckled portions of the several freeways, and collapsed part of the San Francisco-Oakland Bay Bridge.
This video shows how shaking propagated across the Bay Area during the 1989 Loma Prieta earthquake.
Video 9.18: How shaking propagated across the Bay Area during the 1989 Loma Prieta earthquake
If you are using an offline version of this text, access this YouTube video via the QR code.
This video shows destruction caused by the 1989 Loma Prieta earthquake.
Video 9.19: Destruction caused by the 1989 Loma Prieta earthquake
If you are using an offline version of this text, access this YouTube video via the QR code.
9.9.2 Global Earthquakes
Many of history’s largest earthquakes occurred in megathrust zones, such as the Cascadia subduction zone (Washington and Oregon coasts) and Mount Rainier (Washington).
Shaanxi, China (1556): On January 23, 1556, an earthquake of an approximate moment magnitude 8 hit Central China, killing approximately 830,000 people in what is considered the most deadly earthquake in history. The high death toll was attributed to the collapse of cave dwellings (yaodong) built in loess deposits, which are large banks of windblown, compacted sediment (see Chapter 5). Earthquakes in this are region believed to have a recurrence interval of 1,000 years.
Lisbon, Portugal (1755): On November 1, 1755, an earthquake with an estimated moment magnitude range of 8–9 struck Lisbon, Portugal, killing between 10,000 to 17,400 people. The earthquake was followed by a tsunami, which brought the total death toll to between 30,000–70,000 people.
Valdivia, Chile (1960): The May 22, 1960, earthquake was the most powerful earthquake ever measured, with a moment magnitude 9.4–9.6 and lasting an estimated ten minutes. It triggered tsunamis that destroyed houses across the Pacific Ocean in Japan and Hawai’i and caused vents to erupt on the Puyehue-Cordón Caulle (Chile).
Below is a video describing the tsunami produced by the 1960 Chile earthquake.
Video 9.20: Tsunami produced by the 1960 Chile earthquake
If you are using an offline version of this text, access this YouTube video via the QR code.
Tangshan, China (1976): Just before 4 a.m. (Beijing time) on July 28, 1976, a moment magnitude 7.8 earthquake struck Tangshan (Hebei Province), China, and killed more than 240,000 people. The high death toll is attributed to people still being asleep or at home and most buildings being made of unreinforced masonry.
Sumatra, Indonesia (2004): On December 26, 2004, slippage of the Sunda megathrust fault generated a moment magnitude 9.0–9.3 earthquake off the coast of Sumatra, Indonesia. This megathrust fault is created by the Australia plate subducting below the Sunda plate in the Indian Ocean. The resultant tsunamis created massive waves as tall as 24 m (79 ft) when they reached the shore and killed more than an estimated 200,000 people along the Indian Ocean coastline.
Haiti (2010): The moment magnitude 7 earthquake that occurred on January 12, 2010, was followed by many aftershocks of magnitude 4.5 or higher. More than 200,000 people are estimated to have died as result of the earthquake. The widespread infrastructure damage and crowded conditions contributed to a cholera outbreak, which is estimated to have caused thousands more deaths.
Tōhoku, Japan (2011): Because most Japanese buildings are designed to tolerate earthquakes, the moment magnitude 9.0 earthquake on March 11, 2011, was not as destructive as the tsunami it created. The tsunami caused more than 15,000 deaths and tens of billions of dollars in damage, including the destructive meltdown of the Fukushima nuclear power plant.
Take this quiz to check your comprehension of this section.
If you are using an offline version of this text, access the quiz for Section 9.9 via the QR code.
Summary
Geologic stress, applied force, comes in three types: tension, shear, and compression. Strain is produced by stress and results in three types of deformation: elastic, ductile, and brittle. Geological maps are two-dimensional representations of surface formations, which are the surface expression of three-dimensional geologic structures in the subsurface. The map symbol called strike and dip, or rock attitude, indicates the orientation of rock strata with reference to north-south and horizontal. Folded rock layers are categorized by the orientation of their limbs—fold axes and axial planes. Faults result when stress forces exceed rock integrity and friction, leading to brittle deformation and breakage. The three major fault types are described by the movement of their fault blocks: normal, strike-slip, and reverse.
Earthquakes, or seismic activity, are caused by sudden brittle deformation accompanied by elastic rebound. The release of energy from an earthquake focus is generated as seismic waves. P and S waves travel through the Earth’s interior. When they strike the outer crust, they create surface waves. Human activities, such as mining and nuclear detonations, can also cause seismic activity. Seismographs measure the energy released by an earthquake using a logarithmic scale of magnitude units; the moment magnitude scale has replaced the original Richter scale. Earthquake intensity is the perceived effects of ground shaking and physical damage. The location of earthquake foci is determined from triangulation readings from multiple seismographs.
Earthquake rays passing through rocks of the Earth’s interior and measured at the seismographs of the worldwide Seismic Network allow 3-D imaging of buried rock masses as seismic tomographs.
Earthquakes are associated with plate tectonics. They usually occur around the active plate boundaries, including zones of subduction, collision, and transform and divergent boundaries. Areas of intraplate earthquakes also occur. The damage caused by earthquakes depends on a number of factors, including magnitude, location and direction, local conditions, building materials, intensity and duration, and resonance. In addition to damage directly caused by ground shaking, secondary earthquake hazards include liquefaction, tsunamis, landslides, seiches, and elevation changes.
Take this quiz to check your comprehension of this chapter.
If you are using an offline version of this text, access the quiz for Chapter 9 via the QR code.
Text References
- Christenson, G.E. (1995). The September 2, 1992, ML 5.8 St. George earthquake, Washington County, Utah. Utah Geological Survey Circular 88.
- Coleman, J.L., and Cahan, S.M. (2012). Preliminary catalog of the sedimentary basins of the United States. United States Geological Survey Open-File Report 1111.
- Earle, S. (2015). Physical geology OER textbook: BC Campus OpenEd.
- Feldman, J. (2012). When the Mississippi ran backwards: Empire, intrigue, murder, and the New Madrid earthquakes of 1811 and 1812: Free Press.
- Fuller, M.L. (1912). The New Madrid earthquake. Central United States Earthquake Consortium Bulletin 494, 129.
- Gilbert, G.K., and Dutton, C.E. (1877). Report on the geology of the Henry Mountains: U.S. Government Printing Office, 160.
- Hildenbrand, T.G., and Hendricks, J.D. (1995). Geophysical setting of the Reelfoot rift and relations between rift structures and the New Madrid seismic zone. United States Geological Survey Professional Paper 1538-E, 36.
- Means, W.D. (1976). Stress and strain – Basic concepts of continuum mechanics: Springe.
- Ressetar, R. (ed.). (2013). The San Rafael Swell and Henry Mountains Basin: Geologic centerpiece of Utah. Utah Geological Association, 250.
- Satake, K., and Atwater, B.F. (2007). Long-term perspectives on giant earthquakes and tsunamis at subduction zones. Annual Review of Earth and Planetary Sciences, 35(1), 349–374. doi: 10.1146/annurev.earth.35.031306.140302.
- Talwani, P., and Cox, J. (1985). Paleoseismic evidence for recurrence of Earthquakes near Charleston, South Carolina. Science, 229(4711), 379–381.
Figure References
Figure 9.1: Types of stress. Michael Kimberly, North Carolina State University via United States Geological Survey (USGS). 2021. Adapted by Laura Neser. Public domain. https://www.usgs.gov/media/images/stresstypesgif
Figure 9.2: Different materials deform differently when stress is applied. Steven Earle. 2019. CC BY. Figure 12.1.1 from https://opentextbc.ca/physicalgeology2ed/chapter/12-1-stress-and-strain
Figure 9.3: “Strike” and “dip” are words used to describe the orientation of rock layers with respect to north/south and horizontal. CrunchyRocks. 2018. CC BY 4.0. https://commons.wikimedia.org/wiki/File:Strike_and_dip_on_bedding.svg
Figure 9.4: Attitude symbol on geologic map (with compass directions for reference) showing strike of N30°E and dip of 45° to the SE. Kindred Grey. 2022. CC BY 4.0. Includes Compass Rose by NAPISAH from Noun Project (Noun Project license).
Figure 9.5: Model of anticline. Speleotherm. 2016. CC BY-SA 4.0. https://commons.wikimedia.org/wiki/File:Anticline.png
Figure 9.6: An anticline near Bcharre, Lebanon. Not home. 2005. Public domain. https://commons.wikimedia.org/wiki/File:Anticline-lebanon.jpg
Figure 9.7: Monocline at Colorado National Monument. Anky-man. 2007. CC BY-SA 3.0. https://commons.wikimedia.org/wiki/File:Monocline.jpg
Figure 9.8: This prominent circular feature in the Sahara Desert of Mauritania has attracted attention since the earliest space missions because it forms a conspicuous bull’s-eye in the otherwise rather-featureless expanse of the desert. NASA/GSFC/MITI/ERSDAC/JAROS, and US/Japan ASTER Science Team. 2000. Public domain. https://commons.wikimedia.org/wiki/File:ASTER_Richat.jpg
Figure 9.9: The Denver Basin is an active sedimentary basin at the eastern extent of the Rocky Mountains. Daniel H. Knepper, Jr. (ed.), USGS. 2002. Public domain. https://commons.wikimedia.org/wiki/File:Denver_Basin_Location_Map.png
Figure 9.10: Common terms used for normal faults. Kindred Grey. 2022. CC BY-SA 3.0. Includes Faults6 by Actualist, 2013 (CC BY-SA 3.0, https://commons.wikimedia.org/wiki/File:Faults6.png).
Figure 9.11: Example of a normal fault in an outcrop of the Pennsylvanian Honaker Trail Formation near Moab, Utah. James St. John. 2007. CC BY 2.0. https://commons.wikimedia.org/wiki/File:Faults_in_Moenkopi_Formation_Moab_Canyon_Utah_USA_01.jpg
Figure 9.12: Faulting that occurs in the crust under tensional stress. USGS; adapted by Gregors. 2011. Public domain. https://commons.wikimedia.org/wiki/File:Fault-Horst-Graben.svg
Figure 9.13: Simplified block diagram of a reverse fault. Kindred Grey. 2022. CC BY-SA 3.0. Includes Faults6 by Actualist, 2013 (CC BY-SA 3.0, https://commons.wikimedia.org/wiki/File:Faults6.png).
Figure 9.14: Terminology of thrust faults (low-angle reverse faults). Woudloper. 2006. Public domain. https://commons.wikimedia.org/wiki/File:Thrust_system_en.jpg
Figure 9.15: Thrust fault in the northern Qilian Mountains (Qilian Shan). Jide. 2006. CC BY-SA 3.0. https://commons.wikimedia.org/wiki/File:Thrust_fault_Qilian_Shan.jpg
Figure 9.16: Flower structures created by strike-slip faults. Mikenorton. 2009. CC BY-SA 3.0. https://commons.wikimedia.org/wiki/File:Flowerstructure1.png
Figure 9.17: Process of elastic rebound: (a) Undeformed state, (b) accumulation of elastic strain, and (c) brittle failure and release of elastic strain. Steven Earle. Unknown date. CC BY 4.0. Figure 11.2 from https://open.maricopa.edu/physicalgeology/chapter/11-1-what-is-an-earthquake
Figure 9.18: The hypocenter is the point along the fault plane in the subsurface from which seismic energy emanates. Derived from original work by Sam Hocevar. 2014. CC BY-SA 1.0. https://commons.wikimedia.org/wiki/File:Epicenter_Diagram.svg
Figure 9.19: Example of constructive and destructive interference. Note the red line representing the results of interference. Lookangmany, thanks to author of original simulation, Wolfgang Christian, and to Francisco Esquembre, author of Easy Java Simulation. 2015. CC BY-SA 4.0. https://commons.wikimedia.org/wiki/File:Waventerference.gif
Figure 9.20: P waves are compressional. Christophe Dang Ngoc Chan. 2006. CC BY-SA 3.0. https://commons.wikimedia.org/wiki/File:Onde_compression_impulsion_1d_30_petit.gif
Figure 9.21: S waves are shear. Christophe Dang Ngoc Chan. 2006. CC BY-SA 3.0. https://commons.wikimedia.org/wiki/File:Onde_cisaillement_impulsion_1d_30_petit.gif
Figure 9.22: Frequency of earthquakes in the Central United States. USGS. 2019. Public domain. https://commons.wikimedia.org/wiki/File:Cumulative_induced_seismicity.png
Figure 9.23: A seismogram showing the arrivals of the P, S, and surface waves. Kindred Grey. 2022. CC BY 4.0. Adapted from USGS (Public domain, https://www.usgs.gov/media/images/seismic-wave-showing-p-wave-and-s-wave-initiation).
Figure 9.24: Global network of seismic stations. USGS. 2022. Public domain. https://www.usgs.gov/media/images/global-seismographic-network-gsn-stations
Figure 9.25: Speed of seismic waves with depth in the Earth. Brews ohare. 2010. CC BY-SA 3.0. https://commons.wikimedia.org/wiki/File:Speeds_of_seismic_waves.png
Figure 9.26: Simplified and interpreted P- and S-wave velocity variations in the mantle across southern North America, showing the subducted Farallon plate. Oilfieldvegetarian. 2016. CC BY-SA 4.0. https://commons.wikimedia.org/wiki/File:FarallonTomoSlice.png
Figure 9.27: Tomographic image of the Farallon plate in the mantle below North America. Stuart A. Snodgrass and Hans-Peter Bunge via NASA. 2002. Public domain. https://commons.wikimedia.org/wiki/File:Farallon_Plate.jpg
Figure 9.28: Example of a shake map. USGS. 2012. Public domain. https://en.wikipedia.org/wiki/File:USGS_Shakemap_-_1979_Imperial_Valley_earthquake.jpg
Figure 9.29: Example of devastation on unreinforced masonry by seismic motion. M. Mehrain, Dames, and Moore via NOAA/NGDC. 2012. Public domain. https://commons.wikimedia.org/wiki/File:Collapse_of_Unreinforced_Masonry_Buildings,_Iran_(Persia)_-_1990_Manjil_Roudbar_Earthquake.jpg
Figure 9.30: Fault trench near Teton Fault. Jaime Delano via USGS. 2017. Public domain. https://www.usgs.gov/media/images/teton-fault-4
Figure 9.31: High density of earthquakes in the New Madrid seismic zone. Kbh3rd. 2011. CC BY-SA 3.0. https://commons.wikimedia.org/wiki/File:New_Madrid_Seismic_Zone_activity_1974-2011.svg
Figure 9.32: Buildings toppled from liquefaction during a 7.5 magnitude earthquake in Japan. Ungtss. 1964. Public domain. https://commons.wikimedia.org/wiki/File:Liquefaction_at_Niigata.jpg
Figure 9.33: As the ocean depth becomes shallower, the wave slows down and piles up on top of itself, making large, high-amplitude waves. Régis Lachaume. 2005. CC BY-SA 3.0. https://commons.wikimedia.org/wiki/File:Propagation_du_tsunami_en_profondeur_variable.gif
Figure 9.34: Schoolhouse in Thistle, Utah, destroyed by a landslide. Jenny Bauman. 2006. CC BY-SA 2.0. https://commons.wikimedia.org/wiki/File:Thistle-School_house.jpg
Figure 9.35: Remains of San Francisco after the 1906 earthquake and fire. Lester C. Guernsey. 1906. Public domain. https://commons.wikimedia.org/wiki/File:San_Francisco_1906_earthquake_Panoramic_View.jpg
Figure Descriptions
Figure 9.1: Tensional stress where dominant stresses are pulling away from the object, compressional stress where dominant stress is pushing in towards the object, and shear, where part of the object is pushed and part of the object is pulled (stresses in opposite directions)
Figure 9.2: Chart with Stress increasing upward and Strain increasing toward the right; three color-coded curves are on the graph: curve A is green and increases upward with little movement toward the right until it ends near the top; curve B is red and increases upward before abruptly ending; and curve C is yellow and has a broad arc up and to the right before ending toward the far right of the chart.
Figure 9.3: Block diagram showing three stacked sedimentary layers gently dipping with a transparent horizontal surface overlain on top of the layers; there is a dashed line where the horizontal layer intersects the dipping layers with the label Horizontal line on the dipping surface; the angle between the horizontal layer and the dipping layers is 20 degrees and labeled Dip; on the top of the dipping layers is a T-shaped symbol labeled Strike and dip symbol, with the number 20 in the lower right. In the upper right of the figure is a T-shaped symbol with an arrow along the longest part labeled Strike Direction; at the lower right of the T is the number 20, labeled Dip angle; and the smaller part of the symbol is labeled Indicates dip direction (downhill).
Figure 9.4: A compass rose showing N, E, S, and W; a T-shaped strike and dip symbol is next to it with the longest part oriented roughly northeast-southwest. The shortest part of the strike and dip symbol points toward the southeast and is labeled 45 degrees.
Figure 9.5: Model of anticline. Oldest beds are in the center and youngest on the outside. The axial plane intersects the center angle of bend. The hinge line follows the line of greatest bend, where the axial plane intersects the outside of the fold; two arrows point inward toward the axial plane labeled Stress.
Figure 9.6: View of a hillside with exposed sedimentary layers that form an arch shape.
Figure 9.7: View of a cliffside with a series of tan to red sedimentary layers visible; they are horizontal on the left-hand side and angle downward toward the right.
Figure 9.8: View of a dome from space. The upwarped beds of rock form concentric circles, where the center of the dome has been eroded away.
Figure 9.9: Schematic map of the Denver Basin, a sedimentary basin under under northeastern Colorado, southeastern Wyoming, and southwestern Nebraska; the Front Range Uplift runs approximately north to south along the left side of the basin. The map includes a cross section of the area, showing yellow sedimentary beds folding downward into a syncline.
Figure 9.10: Block diagram of a normal fault. The footwall is the block on the left which has moved upward and the hanging wall is the block on the right which has moved downward; a fault runs from upper left to lower right between the blocks; the exposed side of the foot wall along the surface is labeled Fault scarp.
Figure 9.11: Roadcut cliffside outcrop of multicolor sedimentary beds offset by a normal fault; on either side of the fault, the rocks on the right-side hanging wall have moved downward relative to the rocks on the left-side foot wall.
Figure 9.12: Block diagram showing a series of three uplifted horsts with a downthrown graben between each horst; the entire area extends with arrows pointing outward; there are normal faults at each of the contacts between horsts and grabens.
Figure 9.13: Block diagram of a reverse fault. The hanging wall is the block on the left which has moved upward and the footwall is the block on the right which has moved downward; a fault runs from upper right to lower left between the blocks.
Figure 9.14: Block diagram of a landscape with numerous thrust fault features: the base of the landscape is the footwall, labeled Autochton material and colored white; on the left-hand side of the diagram, a gray oval-shaped piece of land is on top of the landscape with a thrust fault at its base, labeled klippe; gray hills sit on top of the white landscape on the right-hand side of the diagram with a thrust fault at its base, labeled Nappe; there is a white oval-shaped exposure in the gray hills labeled Window.
Figure 9.15: View of a cliffside with gray rocks on the left-hand side; red rocks are to the right of the gray rock with a fault plane visible below the red rocks, angled from the lower left to upper right; below and on the right side of the fault are brown sedimentary rocks.
Figure 9.16: Two block diagrams: the left diagram shows mountains that have formed on the surface as a result of a strike-slip fault causing wedges of rock to move upward, labeled Positive Flower; the right diagram shows basins that have formed on the surface as a result of a strike-slip fault causing wedges of rock to move downward, labeled Negative Flower.
Figure 9.17: A (prestressed state): rectangle with no movement. B (stressed rock deforms elastically): rectangle has curvy movement in the middle from top being pulled left and bottom being pulled right. C (post-rupture, unstressed rock): rectangle split in the middle on the rupture surface or fault plane. top moves left, bottom moves right.
Figure 9.18: The hypocenter is the point from which seismic energy emanates. The epicenter is the point on land surface vertically above the hypocenter.
Figure 9.19: Animated GIF showing green waves moving toward the right and blue waves of equal amplitude and wavelength moving toward the left; a red wave shows the resulting wave amplitude as the waves interact: when their wave crests match up, the total wave amplitude increases; when one wave crest matches with the other wave trough, the wave amplitude goes to zero.
Figure 9.20: Animated GIF showing a an approximately 30 by 30 grid with a compressional wave traveling through the grid, pushing the vertical lines closer together as it travels.
Figure 9.21: Animated GIF showing a an approximately 30 by 30 grid with a shear wave traveling through the grid, creating s-shaped curves as it travels.
Figure 9.22: A bar graph: the y-axis is “Number of M3+ Earthquakes” with a scale of 0 to 1200, and the x-axis is the year in question with a scale of 1970 to 2020. The bars spike sharply up after the year 2009, with the highest bar peak in 2015. There is also an inset map of the central United States that has dots of locations of earthquakes during this period, color-coded by magnitude. The densest cluster is in Oklahoma.
Figure 9.23: Squiggly lines along a horizontal axis. When the P-wave arrives, a small amplitude squiggle shows up. Then the S-wave arrives, and another small-amplitude squiggle shows. Finally, the surface-waves arrive, and large-amplitude waves show up, two to three times the amplitude of the body waves. Then the wave taper off and the line becomes essentially horizontal again.Number of seconds between the P and S waves is the distance from station to earthquake epicenter.
Figure 9.24: World map showing the distribution of Global Seismographic Network (GSN) stations. USGS GSN site locations are shown as blue triangles and IRIS/IDA station locations are shown as green triangles; the stations are spread across the entire globe.
Figure 9.25: Graph showing velocity of seismic waves in km/s increasing toward the right from 0 to 14 and depth in km increasing downward from 0 to 6000. S-wave is plotted in purple and increases velocity downward from approximately 3 km/s at 0 km depth to 6.5 km/s at 2900 km depth; at 2900 km the S-wave curve goes down to 0 km/s until it reaches 4600 km depth, where it increases to 4 km/s downward from there. P-wave is plotted in brown and increases velocity downward from approximately 6 km/s at 0 km depth to 14 km/s at 2900 km depth; at 2900 km the S-wave curve goes down to 7 km/s and begins increasing downward to 11 km/s at 6000 km depth. On the right-hand side of the diagram is a vertical bar with Mantle labeled at 0 to 2900 km depth, D”-layer labeled at 2900 to 3000 km depth, Outer Core labeled at 3000 to 5100 km, and Inner Core labeled at 5100 km to the bottom of the graph.
Figure 9.26: Two cross sections across the United States with the following color-coded seismic velocities: blue represents faster than average, red represents slower than average, and yellow represents near average. The top cross section is labeled P-wave velocity variations and there is a visible angled slab colored in blue that goes from about 420 km depth down toward the bottom of the diagram at 2700 km depth, labeled subducted Farallon Plate?; there is also blue along the top of the diagram near the center, with red blobs on the left and right near the surface; the rest is colored yellow. The bottom cross section is labeled S-wave velocity variations and there is a visible angled slab colored in blue that goes from the surface down toward the bottom of the diagram and ends at 2600 km depth, labeled subducted Farallon Plate?; there are small red blobs on the left near the surface as well as toward the right at depths of 400 to 700 km; the rest is colored yellow.
Figure 9.27: 3D yellow sphere centered on North America with red plate tectonic boundaries drawn on the surface; there is a wedge-shaped slice cut out which reveals a blue blobby slab visible at depth, representing the Farallon plate in the mantle.
Figure 9.28: Square shaded relief map centered over the Imperial Valley of southern California with latitude and longitude labeled around the edges of the map. Near the center of the map is star that marks an earthquake epicenter on the edge of a small red area shaded on the map; radiating outward from the red area are lighter red to orange to yellow areas, representing less and less shaking felt away from the epicenter of the earthquake. At the bottom of the figure is a chart showing the Mercalli Scale (described in the text).
Figure 9.29: Color photograph of rubble from the remains of a collapsed building; in the background are vegetation-covered mountains.
Figure 9.30: 5 people in bright vests stand at the bottom of a trench.
Figure 9.31: Map centered on southeastern Missouri, western Kentucky, northwestern Tennessee, southern Illinois, and northeastern Arkansas; earthquake epicenters are marked as red dots with a visible concentration near the border of southeastern Missouri, northwestern Tennessee, and northeastern Arkansas.
Figure 9.32: Three rows of rectangular-shaped buildings with the second and third rows of buildings having fallen over.
Figure 9.33: Animated gif showing large wavelength, low-amplitude waves in the deep ocean and high-amplitude, low-wavelength waves in the shallow ocean. Frequency decreases with depth.
Figure 9.34: Extremely damaged brick structure with only an archway remaining.
Figure 9.35: Panoramic black-and-white photo of rubble and the remains of buildings, some still smoking.
Energy that radiates from fault movement via earthquakes.
Force applied to an object, typically in relation to forces within the Earth.
The deformation that results from application of a stress.
A type of deformation that reverses when the stress is removed.
A bending, squishing, or stretching style of deformation where an object changes shape smoothly.
The point at which the amount of strain on a substance has caused the maximum amount of elastic deformation and switches to ductile deformation.
A style of strain in which an object suddenly breaks, fractures, or otherwise fails in a way different from ductile deformation.
An extensive, distinct, and mapped set of geologic layers.
A measure of a plane's maximum angle with respect to horizontal, where a perfectly horizontal plane has a dip of zero and a vertical plane has a dip of 90°.
A measure of a geologic plane's orientation in 3-D space. Used to measure beds of rocks, faults, foldhinges, and more. Using the right-hand rule, dip is perpendicular and to the right 90° of the strike.
A rock layer that has been bent in a ductile way instead of breaking (as with faulting).
A two-dimensional line that divides the two sides of a fold.
Downward-facing fold that has older rock in its core.
A geologic circumstance (such as a fold, fault, or change in lithology) that allows petroleum resources to collect.
A U-shaped, upward-facing fold with younger rocks in its core.
A one-sided, fold-like structure in which layers of rock warp upwards or downwards.
A basin formed structurally by symmetrical synclines.
A local or regional depression that allows sediments to accumulate.
Faulting that occurs with a vertical motion.
On a dipping fault, the part of the block that is below the fault; moves down in normal faulting, up in reverse faulting.
On a dipping fault, the side that is on top of the fault plane; moves down in normal faulting, up in reverse faulting.
Amount of movement during a faulting event.
A rock surface that has been polished by fault movement, covered with grooves.
A dip-slip fault in which the hanging wall drops relative to the footwall, caused by extensional forces.
A valley formed by normal faulting on just one side.
A style of low-angle, high-extension normal faulting.
A dip-slip fault in which the hanging wall rises with respect to the foot wall.
Referring to high-magnitude faulting that occurs in subduction.
A strike-slip or transform motion in which the relative motion is to the left as viewed across the fault.
Movement in a transform or strike-slip setting toward the right as viewed across the fault.
Stresses that push objects together into a smaller surface area or volume; contracting forces.
Stress that pulls objects apart, giving them a larger surface area or volume; stretching forces.
A small area along a strike-slip or transform fault with branching structures of transpression/transtension, that cause local hills or valleys.
A theory concerning built-up energy that is released during an earthquake.
Initiation point of an earthquake or fault movement.
A slow and steady movement that can occur as part of faults, mass wasting, and grain movement.
Largest earthquake in an earthquake sequence.
Earthquakes that occur after the mainshock, usually decreasing in amount and magnitude over time.
The surface directly above the focus of an earthquake, typically associated with strong damage.
Height or depth of a wave from the middle point.
The distance between any two repeating portions of a wave (e.g., two successive wave crests).
Seismic waves that travel through the Earth, mainly P waves and S waves.
The fastest seismic wave that occurs after an earthquake, compressional in nature.
Second-fastest seismic wave, which has a shear motion.
Waves changing direction due to changing speeds, typically caused by a change in density of the medium.
Seismic waves that only move along the surface, mainly R waves and L waves.
Surface waves that have an up and down motion.
Surface waves that have a side-to-side motion.
Earthquakes that occur due to human activity.
Gaseous fossil fuel derived from petroleum, mostly made of methane.
A process of injecting pressurized fluids into the ground to aid in hydrocarbon migration.
Areas that have an unpredicted change in seismic data, indicating a change in properties.
A measure of earthquake strength. Scales include Richter and moment.
A magnitude scale based on the amplitude of shaking, measured via a seismograph.
A magnitude scale based on calculation of the energy released in an earthquake.
A qualitative earthquake scale of the degree of shaking in an earthquake, ranging from I to XII.
An amplification of earthquake waves due to buildings or other structures.
Length of fault without earthquake activity, occurring due to a locked segment in the fault.
Average time between earthquakes, calculated based on past earthquake records.
Process of saturated sediments becoming internally weak (like quicksand) and destabilizing foundations.
A series of waves produced from a sudden movement of the floor of a ocean basin (or large lake), caused by events such as earthquakes, volcanic eruptions, landslides, and bolide impacts.